Nuclear waste: a comprehensive approach (1)
Waste and nuclear waste: decay periods and potential hazardsSummary
Human activity has reached an unprecedented scale in recent decades in terms of the quantities of materials transformed and the production of waste of all types. Some of this massively produced waste can be reused or recycled. Some is potentially toxic. Discussed here are the characteristics of the potential dangers of nuclear wastes, the physical phenomena at work, and the dangers associated with radioactivity before protection solutions are put into place.
The potential dangers of nuclear waste are comparable to those of other toxic chemical waste produced in large quantities by industry. However, nuclear wastes have the advantage of being present in small quantities (in mass and volume terms) and being very easy to trace. Their quantities and hazardousness are dependent on the recycling strategy adopted.
Jean-Paul Bouttes,
Engineer and economist. Formerly Senior Executive Vice President of Strategy and Prospective and Chief Economist at EDF. Former member of the World Energy Council Studies Committee and Professor of Economics at École Polytechnique.
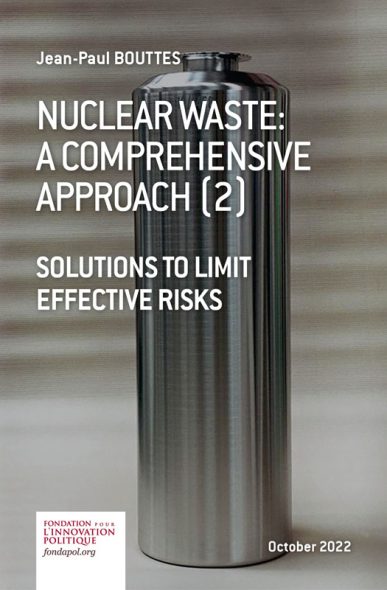
Nuclear waste: a comprehensive approach (2)
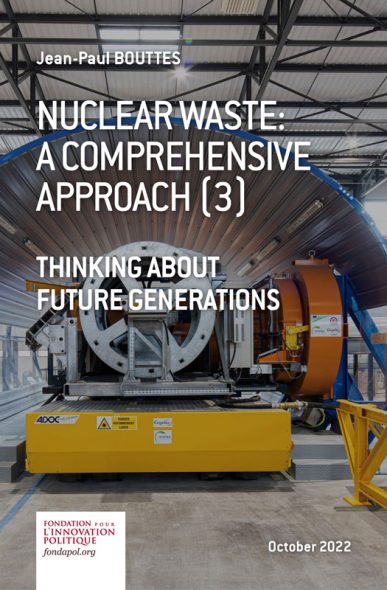
Nuclear waste: a comprehensive approach (3)
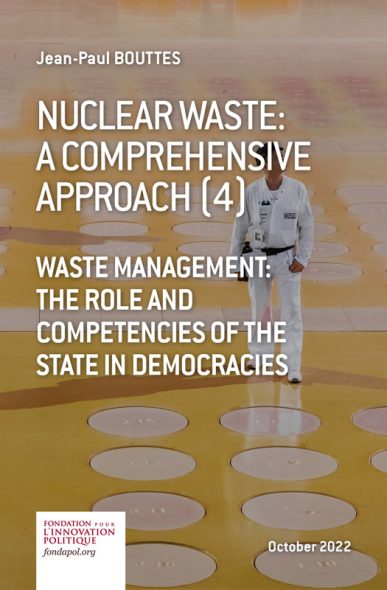
Nuclear waste: a comprehensive approach (4)
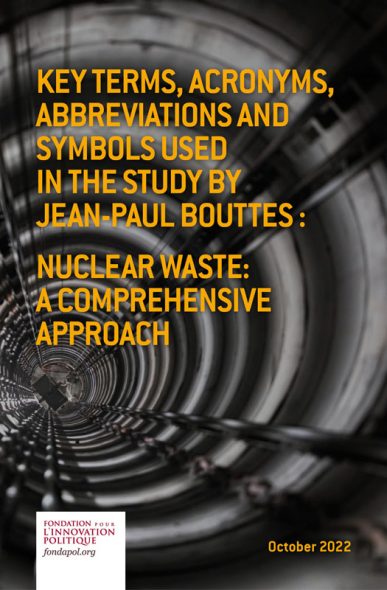
Key terms, acronyms, abbreviations and symbols used in the study by Jean-Paul Bouttes: "Nuclear waste: a comprehensive approach"
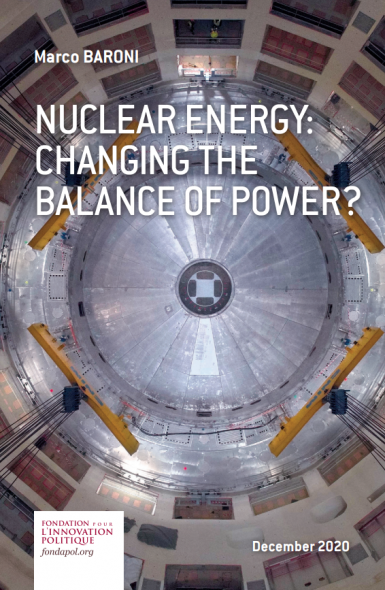
Nuclear energy : changing the balance of power
The author would like to thank the many people who took part in this collective study and made it possible to publish it here, particularly Gilles Bellamy, Bernard Boullis, Lorraine Bouttes, François Dassa, Jean-Michel Delbecq, Antoine Herzog, Daniel Iracane, Roland Masse, Dominique Ochem and Éric Preud’homme for their comments, suggestions and support. The author is solely responsible for the analyses and opinions expressed in this publication. |
Generel Introduction
For more information about French Law 91-1381 of 30 December 1991 relative to radioactive waste management research, named the “Bataille Law” after its rapporteur Christian Bataille, as well as subsequent updates. This law set a December 2006 deadline for the government to submit a report to Parliament providing an overall assessment of research into the future of high-level radioactive waste, along with a bill that could, if necessary, authorise the creation of a repository for that high-level waste.
When energy policy is being debated, wastes associated with civil nuclear power are often discussed in negative terms, to such an extent that they come to be seen as an obstacle to pursuing nuclear programmes. One of the main arguments is that they represent a significant health risk for future generations and a problem for which no satisfactory technological solutions exist. Next, they are presented as a one-of-a-kind challenge due to possible very long-term consequences for future generations and related uncertainties. The argument goes that this creates a moral imperative (“categorical imperative”) to do everything in our power to protect humankind from this waste. Lastly, some say transparency and especially public debate on the subject are not as democratic as they could be. What we propose here is a serious look at each of these three areas of concern, as well as a discussion of nuclear wastes that addresses these different dimensions.
The first set of concerns requires a precise, scientific and technical response to the factual, common-sense questions of citizens: what is the nature and origin of this waste? How much of it is there, what is the decay period, and what are the related health risks over different time horizons? What technological solutions exist to protect ourselves, and how effective are they? Where does our scientific knowledge stand, what uncertainties remain, and what further research is in the pipeline (see Parts II and III)?
To address the second set of concerns, we must look beyond nuclear wastes and consider the broader issue of all the waste humans produce in general and the risks it represents for future generations – toxic industrial waste, production of greenhouse gases, climate change, biodiversity loss… (see Part I). It is only by looking through this wider lens that we can examine the central question of our ethical responsibility to future generations, which we must consider taking into account the capacities of different generations and the nature of the risks to be managed. Our approach must marshal the resources of long-term history and moral philosophies (see Part IV).
Addressing the third set of concerns requires asking what democratic institutions are best suited to meet these relatively recent challenges, which emerged as a result of mankind’s growing mastery of science and technology. By examining the problems encountered both in implementing waste management solutions (see Part V) and conducting public debate and public policymaking processes (see Part VI), we can identify areas where progress would be particularly beneficial in helping future generations tackle much more difficult and important challenges, particularly in terms of changes in the climate system and biodiversity.
Over the course of this journey, we will seek to show that the potential danger posed by nuclear waste is similar to that posed by the toxic waste produced by other parts of the energy sector and industry. However, because nuclear waste is produced in smaller volumes and easy to trace, it is possible to implement confinement solutions that should make the health risks practically negligible, including for future generations. Civil nuclear power is a means of generating electricity with no carbon emissions, and also avoids the production of the many kinds of toxic waste associated with other energy technologies. Moreover, nuclear waste is only one risk – among others – facing future generations. Unlike nuclear waste, some risks are global and irreversible, and there is great uncertainty about their future impact (changes in the climate system and biodiversity, pandemic risk, etc.). Lastly, it should be noted that since 1990, few topics have been the subject of as much public discussion in France as nuclear power – including the debates conducted under the aegis of Parliament with support from the OPECST (Office parlementaire d’évaluation des choix scientifiques et technologiques – French Parliamentary Office for the Assessment of Scientific and Technological Choices) – or as much regulatory control (particularly via French nuclear safety authority ASN).
We will also see major weaknesses revealed in the democratic processes of examining, making decisions about and implementing management solutions – weaknesses that will need to be addressed. Among the thousands of pages of reports that are of high quality from a scientific and technical standpoint, it is difficult to find overviews that are both rigorous and precise, ones that take a pedagogical approach to the fundamental questions citizens have about long-term health impacts, the capacity (in terms of quantities) of potential geological waste disposal facilities, or how nuclear waste risks and management solutions compare to those implemented for toxic industrial waste. The implementation of waste management solutions has been undermined in recent years by policymakers hesitating about which energy strategy to adopt, and by the weakness of the State apparatus in terms of operational capabilities and industrial governance. The solution is to have more multidisciplinary expertise working for States and citizens, to respond to their concerns and enable more efficient industrial implementation. Beyond thinking about nuclear waste management, it would be helpful to create analysis grids for examining the different types of long-term risks society faces, and to engage in ethical reflection about the management of these risks as a whole. This would do a great deal to help us limit the increase of concern about the future: degradation of the environment on our planet, geopolitical risks, questions about the prospects for useful technological innovation… The ultimate goal is to invent forms of cooperation and collective inquiry that allow us to become more proactive, modest and lucid in approaching our collective destiny, but also more effective.
Nuclear waste was one of the first topics explored as a risk potentially affecting future generations, as evidenced by all the research, debates, and decisions implemented as part of the Bataille Law of 19911 on the waste management research strategy. The lessons learned may be particularly useful in tackling challenges that are essential and much more complex (climate, biodiversity), for which we have neither prospective analyses of the risks that will face future generations more than 150 or 200 years from now, nor ethical reflections informed by general risk analysis grids.
Nuclear waste in a society that produces waste
A society that processes materials at a massive scale to produce services and goods generates significant waste
Jacques Varet, “Les ressources minérales : ressources énergétiques et autres”, L’Encyclopédie du développement durable, N°. 45, May 2007, p. 2 (http://encyclopedie-dd.org/IMG/pdf_N-_45_Varet.pdf).
François Grosse, “Économie circulaire”, in Dominique Bourg and Alain Papaux, Dictionnaire de la pensée écologique, PUF, 2015.
The world population is currently estimated at more than 7 billion. It is expected to rise to 10 billion by 2050. Huge quantities of objects and services will have to be produced to meet the needs of these billions of individuals (nutrition, housing, mobility, heating, healthcare, communication, computers, books, musical instruments, etc.), with technological innovations changing how materials are processed along the way. The steady stream of technological innovation we have seen for two centuries now has allowed us to break out of the Malthusian Trap: human life has been improved by all these objects used in the areas of healthcare, nutrition and culture, even as the number of human beings surged. For instance, between 1900 and 2000, while the world population and per-capita energy consumption quadrupled, and energy production rose sixteen-fold, life expectancy at birth nearly doubled.
This evolution has mobilised huge quantities of resources, and massive amounts of waste are generated during the different phases of the extraction-processing-production-use cycle: mining waste produced during extraction, gas or liquid effluents and solid waste produced by processing and production plants, and abandonment of objects (after some are recycled) by users. In the words of Jacques Varet: “In terms of volume displacement, the mineral resources extracted by humans today are having an effect comparable to natural erosion. Extractions of sand and gravel exceed about ten billion tonnes a year. Next on the list are solid rocks, more than 3 billion tonnes of which are extracted annually, and then fossil fuels: coal, oil, gas and lignite2”. In a word, some 40 billion tonnes were already being displaced by humans annually in the early 2000s, representing close to 6t/person/year, compared with about 60 billion tonnes a year due to natural erosion (chiefly rivers and oceans) and 30 billion tonnes due to volcanoes. Moreover, “consumption of steel in the year 2011 – around 1.5 billion tonnes – [was] higher than the combined production of iron by all humans from the prehistoric origins of steelmaking up until the year 19003”.
See The Circularity Gap Report 2020, Circle Economy, 2021, pp. 17-19.
Figures for material consumption by humans in 2020
The Global Circularity Gap Report 2020 includes figures for the world population that are slightly different and interesting*: it found that the global economy was consuming 101 billion tonnes of materials a year, of which 92 billion tonnes were extracted and 9 billion tonnes recycled. Of the total 101 billion tonnes consumed, minerals represented 51 billion tonnes (sand, gravel and non-metallic minerals), metallic minerals about 10 billion tonnes, fossil fuels close to 15 billion tonnes, and biomass 25 billion tonnes. If we subtract the 25 billion tonnes of biomass from the 92 billion tonnes extracted, mineral extraction is close to 67 billion tonnes. In other words, humanity’s impact largely exceeds that of erosion. The report also provides interesting figures related to usage: of the 101 billion tonnes used, just 31 billion were put into long-term stock in the broad sense (net figure: new goods subtracting removals), 22 billion tonnes were dispersed (mostly short-lived consumer goods such as plastics, paperboard, paper, etc.), nearly 15 billion tonnes were emitted into the atmosphere (carbon dioxide equivalent) and 33 billion tonnes were collected as waste, with 9 billion being recycled. Based on these figures, every person on the planet requires about 13t of materials a year (including biomass) and creates about 4.5t of waste, to which we must undoubtedly add 3t dispersed into the environment, bringing the total to around 7.5t per person (representing more than half of waste from all materials consumed). |
Ademe, Déchets chiffres-clés, 2020 edition, Ademe Éditions, September 2020, p. 21.
Where France is concerned, a document published by Ademe in 20204 showed that, factoring in the materials contained in imports (increasingly significant with the offshoring of a large share of extractive and manufacturing activity), close to 25 tonnes of materials were used per person in France in 2018 for domestic consumption. In 2016, France’s non-hazardous waste production was 4.7t per person per year, including 437kg of household waste, 1.5 tonne of “waste generated by economic activities” and 200kg of “hazardous waste” (toxic). In 2017, 326 million tonnes of waste were produced in France, of which 11 million tonnes of “hazardous waste”. These figures take into account waste generated by production and utilisation processes, for which the producer or user has no further need. Indeed, we can intuitively understand waste to be a material that no longer serves a purpose in the foreseeable future for the producer-user, but remains present in a tangible form, usually liquid or solid, and is traced.
It is thus not dispersed by default, or by accident, into the environment. To this waste must be added emissions of greenhouse gases (about 6t/person/year of CO2 in France) and of various local pollutants (SO2, NOx, particles, etc.).
Potential dangers for humans and the environment that make appropriate treatment of materials and wastes necessary
“More than 100,000 chemical substances are used within the European Union, of which 3,000 are produced quantitively around the world […]. Only a few hundred have been the subject of in-depth studies of their toxic properties, and only a small share has undergone formal and quantified assessments of the toxic and ecotoxic risks they represent”. (Christian Ngô and Alain Régent, Déchets, effluents et pollution, impact sur l’environnement et la santé, Dunod, 3rd edition, 2012, p. 125).
See French Academy of Sciences, “Les plastiques dans l’environnement”, 16 March 2021.
Ademe, op. cit., pp. 23-25, 44, 46 and 58.
Material inputs (including natural resources) and wastes may be hazardous, and waste must be treated in an appropriate and well-thought-out way based on its possible impact on humans and the environment.
a) Materials that are both useful and potentially toxic
Many of the material inputs mobilised (resources, intermediate and final products) are useful but can also serve as weapons (chemical or biological), or may be dangerous to humans, non-human living organisms and ecosystems. Examples of this duality include heavy metals (lead, cadmium, mercury), arsenic and cyanide, organochlorines such as dioxins, and organophosphates. These potentially toxic materials are used to make fertilisers, herbicides and pesticides, or are key components in the manufacture of batteries and many other objects.
The hazardousness of these materials, and of certain kinds of waste, varies depending on concentrations and quantities (issue of low doses), as well as on physical forms and associations with other materials. Exposure to the danger can be through contact, inhalation, ingestion or other means. Our natural environment has habituated us to this duality of materials; experience has taught us not to eat the fruit from all trees or taste all mushrooms, for instance, and that we must protect our eyes and skin when handling certain natural elements. The truth is that humans do not invent much – we usually simply imitate what nature has already created, and we observe its laws. Yet we can also find excess in nature, like in humans, and much of what it produces can be dangerous both for living beings and ecosystems, the greater complexity of which often makes it more vulnerable.
b) The increased scale of human activity makes it necessary to think in terms of benefits and risks
What sets the current era apart is the change in scale of human impact on the environment since the two industrial revolutions and after World War II. Humans are today extracting enormous amounts of materials, concentrating them, combining them with others to potentially produce a final product with new properties (explosive, inflammable, etc.). The danger thus resides first in the mines and factories, and later for the consumer; it was for the sake of workers and consumers that protections against these dangers were originally put into place.
The harmful consequences of human activity, augmented by this change in scale, began to manifest through major events and serious accidents: acid rain, the industrial disasters of Seveso and Bhopal, Minamata mercury poisoning, the Love Canal toxic waste disaster, arsenic contamination in the Orbiel Valley, smog pollution in London and Los Angeles… Yet identification of problems associated with hazardous waste and air, water and soil pollution steadily improved. As a result, starting in the 1970s, waste treatment plans were gradually rolled out: reduction of quantities via the recycle-reuse-ecodesign-economy of functionality chain and treatment of what remains taking into account all dangers. A dilute and disperse approach was used to reach relatively low doses, considered to be practically without risk, or else confinement through storage-disposal with appropriate matrices-packaging to prevent risks of explosion or fire. The number and variety of chemical products in question5, and the number of actors involved, make it more difficult to measure and trace these products, and to conduct studies that would give a good idea of the comparative potential dangers of the different substances, and implement and monitor appropriate protective measures.
Approaches that address all these questions (materials consumed, waste produced, dangers associated with processes and products handled) in a more comprehensive and systemic way would help create risk-benefit profiles for the processes involved in producing objects, and to come up with the most satisfactory solution, notably in terms of waste production6. Ademe estimates that of the 11 million tonnes of hazardous waste generated (200kg/person/year in France in 2016), 33% of the 7.2 million tonnes traced ended up in hazardous waste storage facilities at 15 sites in France equipped for surface storage for 30 years or so7.
Some elements are missing from this accounting, which does not trace with accuracy the origin and destination of the different types of toxic waste. Moreover, storage solutions are not thought out beyond the 30-year horizon, even though the waste remains toxic for very long periods and in some cases indefinitely. It would be useful if Ademe offered an overview that was as comprehensive and detailed as the one Andra prepares for nuclear waste, which marked an important step forward in the 2000s, especially with the French National Plan for the Management of Radioactive Materials and Waste (PNGMDR) and Andra’s successive waste inventories. Moreover, it is indispensable that policies governing the treatment of this industrial waste take into consideration the interests of distant future generations.
The specifics of nuclear technology
a) Drawing a distinction between the “worlds” of military and civilian uses, between nuclear reactors and waste
The word “nuclear” refers to physical phenomena that can be used by humans for very different purposes, with possible consequences the nature of which varies greatly as well.
• Military nuclear: the “bomb” concentrates the energy from fission reactions in less than a second, compressing it to increase its destructive effect. It uses nuclear force to create an instantaneous explosion. This is why 80% of the victims killed in Nagasaki and Hiroshima died from the blast effect and fireballs caused by the atomic explosion, and 20% from immediate ionising radiation (powerful γ-rays and neutrons). “Late stochastic effects” associated with the radioactivity of the products dispersed were very low in proportion. These levels of danger are very different from and well above those associated with civil nuclear, be it reactors or waste.
• Civil nuclear: a distinction must be drawn between what happens in a reactor core in operation and as the result of the radioactive decay of waste. As we shall see in more detail below, energy from the nuclear fission of a heavy nucleus – uranium or plutonium – at work in nuclear reactors is 100 to 1,000 times greater than the energy from the radioactive decay of nuclear waste. Additionally, and more importantly, nuclear fission in reactors in operation is sustained by the controlled chain reaction of these fissions over time; fission energy is thus concentrated in years when the reactors are in operation, whereas with waste, the energy is released over hundreds, thousands or even millions of years, depending on the “period” or “half-life” of the radionuclides in the spent fuel.
Energy intensity is thus very different in the two worlds: reactors sustain very powerful physical reactions in their core, under high pressure and temperatures, which is not the case with waste.
The proliferation of nuclear materials that could be used to produce weapons is thus the main danger associated with using nuclear energy, since nuclear weapons, along with some chemical and biological weapons, are the most dangerous on the planet. When it comes to civil nuclear power, verifying reactor safety to avoid serious accidents and limit their impact on workers and local communities is key. It is thus important to develop reactor designs that allow the chain reaction to be controlled (which was, for instance, not the case with the Russian RBMK design in certain configurations, which caused the Chernobyl accident, as did repeated non-compliance with safety instructions) and to ensure that reactor cores cool when stopped, even when operating in degraded mode, for instance after an earthquake or tsunami (which was not the case at Fukushima).
The dangers associated with nuclear proliferation, reactor accidents and waste involve different physical phenomena and are not on the same level. Regarding nuclear waste, after a few decades, the dangers posed to humans and ecosystems are broadly comparable to those posed by chemical or biological waste. In France, responsibility for ensuring that adequate safeguards are in place to limit effective risks is entrusted to nuclear safety authority ASN for radiation protection and nuclear safety, applying the concept of defence in depth. At the international scale, the IAEA notably coordinates the design and implementation of safeguards against proliferation risks (with support from Euratom at the European level).
b) Nuclear is a source of highly concentrated energy that produces waste that is limited in quantity, traceable and easy to confine
Nuclear is a very concentrated energy. It offers considerable energy potential requiring little handling of materials, limited resources (1,000t of fuel a year to produce three-quarters of French electricity), and little space (some 20 industrial sites in France for reactors and the main fuel-cycle facilities). Nuclear produces very little radioactive waste, in mass and volume terms, relative to the energy produced: 1 to 2kg/person/year for all nuclear waste and 10g/person/year for long-lived waste (compared with 100 to 200kg/person/year for toxic industrial waste). The waste is easy to trace since of all physical and chemical phenomena, radioactivity is the one that can be measured best, and with a remarkable degree of precision (using a Geiger counter or spectrometer): every radioactive element creates its own signature with the type of energy/frequency radiation it emits.
This waste is potentially hazardous over what may be a long period, though it becomes less dangerous over time as its radioactivity gradually decreases. It is also easy to confine since suitable techniques already exist involving glass, cement, bitumen matrixes, concrete or steel packages, and near- surface storage (under water or dry) or deep geological disposal facilities.
Moreover, the small number of actors involved in civil nuclear energy makes it possible to organise waste management with oversight by the government and safety authorities, provided that public authorities properly structure their long-term strategies and implementation.
Waste from electricity generation and the energy transition
IEA, “Net Zero by 2050. A Roadmap for the Global Energy Sector”, May 2021.
See Vaclav Smil, Power Density. A Key to Understanding Energy Sources and Uses, The MIT Press, 2015.
French Academy of Sciences, “Considérations sur les réacteurs nucléaires du futur”, 14 June 2021, p. 15.
Ibid., p. 17.
See IEA, “The Role of Critical Minerals in Clean Energy Transitions”, May 2021, p. 7.
French Academy of Sciences, “Considérations sur les réacteurs nucléaires du futur”, op. cit., p. 21.
a) The transition to carbon-free electricity addresses the challenges of the coming decades
The quantities of materials, objects and waste extracted, processed and produced by humanity have increased spectacularly in recent decades (during the “30 Glorious Years” and then with the emergence of developing countries: China, India, Brazil, South Africa). Now society is facing new challenges and changes in scale. Indeed, needs will be considerable over the next century as the global population is expected to grow by about 50% (from 7 billion today to around 10-11 billion toward 2050), knowing that a very large part of humanity has yet to benefit from, but legitimately aspires to, the progress made in terms of healthcare, housing, nutrition and education. The new digital technologies, new materials and biotechnologies that could be developed will produce new objects and new waste. They will also require energy sources that can keep up with growing needs. Lastly, efforts to fight climate change and limit greenhouse gas emissions will accelerate the obsolescence of many objects that will need to be replaced by others that produce or use carbon-free energy, notably electricity which is, for many uses, the simplest form of energy for producing without emitting greenhouse gases. These different challenges and issues all make an energy transition critical.
That energy transition will, on the one hand, drive up electrification of uses (electric vehicles, heat pumps, etc.) and the production of other decarbonised energy carriers like hydrogen; on the other hand, it will involve substituting carbon-free electricity for fossil fuels to work toward the “net zero emissions” target by 2040, or by 2050 for the global electricity sector. The orders of magnitude of new electricity needs and changes in how that electricity is produced, alluded to in studies from the IPCC, IEA and WEC, are considerable. One IEA study includes a scenario in which, by 2050, electricity use rises by a factor of 2.6 and the global electricity mix changes from two-thirds fossil fuels today (of which 40% coal and 20% gas) to one in which solar makes up 33% (photovoltaic panels) and wind power 35%, with the two carbon-free “dispatchable” sources accounting for roughly the same shares as today (hydropower and nuclear, respectively 12% and 8% of the mix in 2050), though this would still imply a doubling or trebling of their installed capacity8. It is important to underscore both the magnitude of the shift – of the order of geoengineering the surface area of the planet – and the speed at which the changes will take place. Questions thus arise about how ecosystems can adapt to such changes in such a short time, and whether these scenarios fully take into account the multiple impacts and systemic effects of these massive changes (today’s solutions may be tomorrow’s problems). The IEA scenario calls for intermittent energy sources, the density of which is lower than with fossil fuels and much lower than for nuclear, to make up 70% of the mix, with their low density posing two difficulties in particular: use of land and use of materials.
b) Competition over land use will become more intense
Studies have recently begun to focus on issues around the space required for the massive deployment of these energy sources that are carbon-free but have very low density. They require large-scale development of grids to carry power to consumers, as well as backup generation sources and daily, weekly and seasonal storage solutions to ensure that supply and demand match at all times. The space taken up by fossil fuel or nuclear power plants today is an order of magnitude of 1,000 lower that the land currently used for agriculture, livestock farming, forests, and urbanisation9. The land used for solar and wind power would be 100 to 1,000 times greater on a per kilowatt hour basis than for nuclear power. The French Academy of Sciences estimates that generating power equivalent to the output of one pressurised water reactor (PWR), i.e. 1,000 MWe, or 7.44 TWh/year, would require 2,000 wind turbines with a capacity of 2 MWe each (about 150 metres tall), i.e. 200 farms with ten units each, or 620 solar farms (each with 24,000 panels) sitting on 15,500 ha, which represents the surface area of the city of Paris10. To produce the equivalent of 400 TWh, the annual output of the 19 nuclear power plants in France, it would take about 50 times this 7.44 TWh output, meaning that same surface area would be occupied in more than half of all French departments.
Competition for land use would thus become more intense, as would the consequences for biodiversity of a massive deployment of wind turbines and solar panels in rural areas, including some that have heretofore been relatively protected from human activity (and these figures do not take into account the surface area required for backup generation, storage facilities and power grids).
c) Materials and waste associated with the electricity produced: the need for a full assessment of each technology, including renewables
When materials are sourced and chemically processed for the development of renewable energy sources (wind turbines, solar panels, batteries, electrolysers, medium- and high-voltage networks), effluents and waste are produced, in quantities that increase with lower density energies.
The materials required for these energy sources can be divided into two categories:
– Structural materials, including for civil engineering materials (cement, concrete, steel), common materials (steel, copper, aluminium, glass, plastics, silicon, to which can be added fossil fuels, coal, oil and gas); and
– Strategic materials such as rare earths (which are notably used to produce permanent magnets for wind turbines), the content of which in the Earth’s crust is lower, between a few tenths and a few tens of parts per million (ppm). The content of uranium and thorium is on average comparable to rare earth elements, i.e. about two to three ppm for the former and three times more for the latter.
Building nuclear power plants requires more concrete (factor of two to three) than building fossil fuel power plants with CCS, and especially more steel (factor of five), to ensure safety in the event of an earthquake, airplane crash or other disaster. The construction of solar and wind power plants requires, on a per-megawatt basis, 10 to 20 times more structural materials than the nuclear power plants that exist today, and 10 to 100 times more strategic materials11.
The IEA emphasises the change of scale in the quantities of key critical minerals (lithium, nickel, cobalt, manganese, graphite, rare earths, copper, aluminium) needed for electrification and the deployment of renewable energy sources12. The energy sector will become the biggest consumer of these minerals within a few decades, with its share of consumption for instance rising from 10% to 40% for rare earths, from 20% to 90% for lithium, and from less than 5% to 60% for nickel between 2010 et 2040.
As the French Academy of Sciences writes: “For 2,000 wind turbines or 14 million solar panels to produce as much electricity as a 1GWe PWR over 30 years, some 10 million tonnes of minerals must be processed to extract the materials required to build them. By comparison, over 30 years, a 1 GWe PWR will require the extraction of around 5,000 tonnes of natural uranium, implying the processing of 500,000 tonnes of mineral at 1% grade. Even factoring in the materials required for construction, overall, a PWR requires less mining operations than its equivalent in wind turbines or solar panels13”.
Based on these assessments, a full analysis of the materials used and chemical treatment processes involved must be conducted to allow a comparison of the quantity and potential dangers of the waste produced by different electricity generation technologies in relation to the electricity they yield.
Much of this work has already been done for fossil fuels, hydropower and nuclear electricity. Yet no equally rigorous and systemic study has been conducted on renewable energy sources ensuring the same energy output (for instance, 1 MWh throughout the year). These analyses also need to reflect the massive and rapid changes the climate objectives call for, and the questions those changes would raise in terms of feasibility and potential impacts.
These observations suggest that nuclear power, thanks to its considerable energy density, could have a place in the range of options considered as long as nuclear waste is viewed through the broader lens of waste in general and the consequences for land use and biodiversity. The next step is therefore to look more closely at the volumes and potential dangers of nuclear waste and the management solutions available, before returning to this global issue.
Radioactive waste from civil nuclear : quantities and potential dangers over different time horizons
Key lessons from Part II
Radioactivity and health
• The nuclear phenomena that set off processes involving atomic nuclei (nuclear fusion-fission, chain reaction, radioactive decay of unstable nuclei over time and related α, β, γ, X radiation) are a core part of the functioning of nature, galaxies, the stars and life on Earth.
• The energy intensity, and thus the potential dangers of these nuclear phenomena, are fundamentally different depending on whether one is looking at the impact of the explosion of a nuclear bomb, which is extremely destructive by design (blast effect, shock wave, flash and fires); an accident at a functioning nuclear power plant (chain fission reaction under high pressure and temperature), requiring vigilance on the part of the industrial operator; or radiation associated with the decay over time of radioactive nuclei (α, β, γ), which are an integral part of our everyday environment.
• Much of the Earth’s internal heat comes from the decay of four long-lived radioactive elements present in the Earth’s crust: uranium 238 (4.5 billion years), thorium 232 (14 billion years), uranium 235 (700 million years) and potassium 40 (1.25 billion years). This heat, together with that of the Sun, has supported the development of life and ecosystems on Earth.
• The health consequences of ionising radiation (α, β, γ, X) relate to the capacity of its energy to cause ionisation of the air, biological molecules or water molecules, creating within living tissues free radicals (H , OH and “oxidation”) that will react with other molecules and affect the life of the cell and its DNA. These molecular lesion mechanisms, which can be carcinogenic, result from oxidative stress also triggered by numerous carcinogenic chemical toxins, outside of other specific attack pathways: adducts and disruption of enzymatic mechanisms as observed with cadmium and arsenic. These consequences vary depending on the doses that end up in the body and reach sensitive organs, and on how long they are retained within those organs.
Waste from energy production: quantities and potential dangers
• Theradioactivityofnuclearwastestemsprimarilyfromthespentfuel,i.e. fuel that has been “burnt” in the reactor. These quantities are small, in mass and volume terms, especially if the 95% of the fuel – uranium and plutonium – that was not used is recycled. What remains in that case is 50t/year of high-level waste – fission products (“ashes” from the nuclear reaction) and minor actinides – which works out to 1g/person/year (compared with 100 to 200kg/person/year for chemical toxic waste).
• The most dangerous radioactive wastes (high-level) are fission products with very short half-lives (a few hours, weeks or years) such as iodine 131 (half-life of eight days). In other words, it is during the first years or decades after the waste leaves the reactor that protection is most important, especially against external irradiation by penetrating γ-rays. After that, between 30 and 300 years, their activity (number of disintegrations per second) is dominated by short-lived fission products such as caesium 137 (half-life of 30 years), the radioactivity of which is reduced by a factor of 1,000 over 300 years (here again, γ and external irradiation), and by a few β-emitters like strontium 90 (half-life of 28.5 years), which is dangerous in the event of internal contamination.
• The level of activity of waste with a very long decay period of more than a million or a billion years is by definition low. When thinking about future generations, it is therefore important to pay special attention to “long-lived intermediate-level waste”, the activity of which declines gradually but slowly, over a few thousands, tens of thousands or hundreds of thousands of years:
– Long-lived fission products like technetium 99 (213,000 years) are responsible for the bulk of residual dangers from external irradiation (including soft β-rays and related X-rays), and some are mobile and water-soluble;
– Minor actinides such as americium 241 (432 years), to which must be added plutonium 239 (24,000 years) if not recycled, represent the greatest threat over the medium term if they are inhaled or ingested by humans and reach sensitive organs. In the latter case, they are poisonous if they make their way into the food chain, like some heavy metals and arsenic, for instance, though they are not very mobile or soluble and are found in very small quantities.
• A study conducted by the IRSN (French Institute for Radiological Protection and Nuclear Safety) on the health impacts for future generations over the medium to long term (300-1,000 years) of an abandoned glass package helps illustrate the benefits of comparing the potential dangers of nuclear waste and toxic industrial waste. As is the case with the latter, the potential dangers associated with nuclear waste are limited and local, but significant for those affected. Hence the importance of planning for very long-term confinement.
The origin of nuclear waste: the functioning of reactors and the fuel cycle chain
To read more on this topic, see Dominique Grenêche, Histoire et techniques des réacteurs nucléaires et de leurs combustibles, EDP Sciences, 2016.
On this topic, see Paul Bonche (ed.), Le Nucléaire expliqué par des physiciens, EDP Sciences, 2002, p. 150-154, and Louis Patarin (ed.), Le Cycle du combustible nucléaire, EDP Sciences, 2002, p. 33.
Real possibilities also exist for 1,000-year durability within the nuclear industry with the use of thorium in molten salt reactors or simply by tapping the immense reservoir of natural uranium in the sea, the production of which is currently limited only by the cost of extracting it.
a) The principle of natural radioactivity
An atom contains negatively charged electrons “in orbit” around a nucleus made of positively charged protons as well as electrically neutral neutrons. Each element of Mendeleev’s Periodic Table is characterised by its number of protons, which matches its number of electrons (atomic number): hydrogen is 1, carbon 6, oxygen 8, iron 26, iodine 53, caesium 55, radium 88, uranium 92, plutonium 94, and americium 95. One chemical element can have several isotopes depending on the number of neutrons in the nucleus. Protons and neutrons of equal mass make up most of the mass of the atom (the mass of electrons is negligible relative to that of the particles constituting the nucleus), their sum constituting the mass number of the isotope: uranium with a mass number of 235 has 235 – 92 = 143 neutrons, plutonium 239 has 145, carbon 12 has six, oxygen 16 has eight. To keep the nucleus of an isotope stable and prevent the positively charged protons from repelling one another too much, the right quantity of neutrons must be present – roughly the same number as protons for the light atoms from helium to iron; from there, approximately in the middle of the periodic table, the share of neutrons must increase gradually relative to the number of protons.
The atomic nuclei of elements from hydrogen to the middle of the periodic table (around iron) were formed by nature, via successive fusions of the basic elements identified in particle physics (quarks, electrons, protons, neutrons…), when the galaxies and stars were being formed. The heaviest – up to uranium – are the product of the later evolution of the life of stars through to the explosions of supernovas. Our planet thus inherited the primary constituents of this “star factory”. This history helps explain the massive presence of hydrogen, iron, and elements that exist but in smaller quantities in the Earth’s crust as well as in the oceans, such as uranium and thorium. Some of these elements have not had time to find their stable structure: they may be too heavy (in the highest mass numbers around uranium) and will eventually lose a bit of their mass by ejecting a helium 4 nucleus (two protons and two neutrons), also called an alpha (α) particle. They may also have too many neutrons, which turn into protons with the emission of an electron (and an antineutrino), referred to as beta (β) radioactivity.
These radioactive disintegrations are at times accompanied by electromagnetic gamma (γ) rays that are very high frequency and high energy (more than X-rays of the same nature, but originating from peripheral electrons). The electrons emitted by β radioactivity may be slowed, and their trajectory deviated when they pass near a nucleus, in which case a “braking photon” is emitted; this is what is known as “braking radiation” (Bremsstrahlung), the origin of the X-rays associated with this β radioactivity, particularly for certain long-lived fission products such as technetium 99. A radioactive element (for instance carbon 14, used to date archaeological discoveries) is characterised by its half-life, or period, which refers to the time it takes for half the atoms comprising the sample to disintegrate (in the case of carbon 14, 5,730 years). Radioactivity is characterised by an exponential decrease in the pace of decay over time: disintegrations are reduced by a factor of two with each period, and thus decrease by a factor of 1,000 after ten periods (210) and by a factor of 1 million after 20 periods. These periods, like the characteristics of α, β and γ radiation with their respective energies, are among the best understood and measured in physics, which explains the interest in various uses for radioactivity, from medicine to industry to archaeology.
b) Energy associated with nuclear fission14
After natural radioactivity was discovered in the late 19th century (by Henri Becquerel in 1896, with Pierre and Marie Curie doing more research after him), in the 1930s, Irène and Frédéric Joliot-Curie discovered a way to create new radioactive isotopes by firing particles (helium nuclei, neutrons, protons) into certain elements (artificial radioactivity). Toward the end of the 1930s, research conducted by Otto Hahn and Lise Meitner in Berlin and Frédéric Joliot, Lew Kowarski and Hans von Halban in Paris would pave the way for nuclear fission and controlled chain reactions by applying the laws of nuclear physics (imitating what happens during the life of stars): if a neutron is fired into them, certain elements will capture the neutron and become another radioactive isotope, meaning, if the heavy nuclei are “fissile”, then they will fission, and this fission will be accompanied by the emission of several neutrons that can in turn create a new identical heavy fissile nucleus. When uranium 238 absorbs a neutron, it undergoes two short β disintegrations (23 minutes and 2.3 days), producing plutonium 239. Both plutonium 239 and uranium 235 are fissile, meaning they split into two nuclei (for instance iodine, caesium, strontium or xenon) and two or three neutrons which, under favourable conditions (geometry of the uranium and content in terms of fissile nuclei, temperature, pressure in the heat exchanger and potentially neutron moderator fluid), will be able to continue these chain reactions.
The orders of magnitude in energy terms are close to 200 MeV for fission, 4-7 MeV for α radioactivity, a few tens or hundreds of kiloelectron-volts for β radioactivity, and 0.1 to 3 MeV for γ radioactivity. By comparison, the combustion of a carbon atom only releases around 4 eV. The energy produced when uranium is transformed into two medium-sized nuclei and two or three neutrons, through the kinetic energy of these particles, is considerable since linked to a loss of mass of all the elements post transformation relative to the mass of the original uranium: the change in energy ΔE is equal to this loss of mass Δm multiplied by the speed of light squared, c2. Einstein’s formula allows us to understand why the levels of energy released in the fission reaction are of the order of 1,000 to 100 times greater than α, β or γ radioactivity, and several million times greater than chemical combustions associated with electronic and non-nuclear phenomena. The controlled fission of 1t of uranium 235 is equivalent to the combustion of 2 million tonnes of oil. This is why, with 8,000t of natural uranium (0.7% uranium 235 and 99.3% uranium 238), France produces 1,000t of enriched uranium fuel that contains about 3.5 to 4% uranium 235, and can with this limited quantity produce 400 TWh of electricity that meets three-quarters of the country’s annual power needs.
c) Radioactive waste generated by nuclear power production and the related fuel cycle
Extracting uranium ore from a mine also requires handling all the radioactive descendants of uranium 235 (0.7-billion-year radioactive period) and uranium 238 (4.5 billion years), including radon gas and its descendants. In the majority of cases, the radioactivity emitted by these radioelements is low for neighbouring communities, below 1 mSv/year for an active mine, and a fraction of a millisievert a year for a mine that has been closed and redeveloped. By comparison, average natural radioactivity in France is 2.4 mSv/year (see below). While the numbers are not high, they must be monitored to ensure they are kept at this low level. A mine can especially have a potential impact on the miners who work in it; in particular, exposure to radon and mineral dust must be strictly controlled through appropriate ventilation systems in the tunnels15.
This extraction phase is also the only one during which large quantities of (weakly) radioactive materials with a long half-life are handled, most of it remaining on-site as its content is often between 1 and 2kg of uranium per tonne of mineral ore (though some reservoirs are significantly more favourable, such as Cigar Lake in Canada).
In the past, to produce 72,000t of uranium in France (enough for about ten years of use based on current levels), Cogema would extract 52 million tonnes of ore. However, we shall see that if it becomes possible to use all the uranium 238 contained in that 72,000t thanks to the development of fast neutron technologies, existing resources (currently the stockpile of depleted and reprocessed uranium) would in theory suffice to produce 400 TWh/year for several millennia16.
The natural radioactivity of these combustible materials does not give rise to any radioactive elements other than those resulting from their decay (“descendants”), with an exponential decline in their level of activity over time relative to that of the ore. In other words, a radioactive element will not spontaneously make what is around it radioactive. Most of the radioactivity is thus that generated in the reactor core via controlled fission reactions. In simplified terms, these include:
– The fission of the uranium 235, and to a lesser degree the plutonium 239, which directly generates short- and long-lived fission products (mass numbers 70 to 170) that emit β-γ (isotopes of iodine, caesium, strontium, technetium…);
– The capture of neutrons by uranium 238 (or uranium 235, plutonium 239…), which generates transuranic elements (atomic numbers higher than 92), major actinides (the most numerous, isotopes of uranium and plutonium) and minor actinides (americium, neptunium, curium…). These actinides are mainly α emitters of long-life (with β emissions at times);
– Activation products, isotopes produced by the neutron bombardment of the structural materials present in the reactor vessel (fuel assembly structures, internal structure of the vessel…) or in the primary water of the vessel (cobalt, nickel, niobium, zirconium, chlore, tritium…).
Categories of waste: concentrated energy means limited quantities, especially of HLW and ILW-LL
a) Categories of radioactive waste
Radioactive wastes are classified first based on their initial radioactivity, roughly reflecting the number of disintegrations per unit of mass (Bq/g), and thus the nature of the substances they contain and their concentration; and second based on their decay period (radioactive period), which expresses the time period during which the substances contained in the waste will remain significantly radioactive.
Radioactivity levels can be classified as very low, low, intermediate or high, and waste can be classified as short-lived, with a radioactive period of less than 31 years, or long-lived, if the decay period is 31 years or longer.
Table 1: Categories and cumulative quantities of nuclear waste in France at the end of 2019
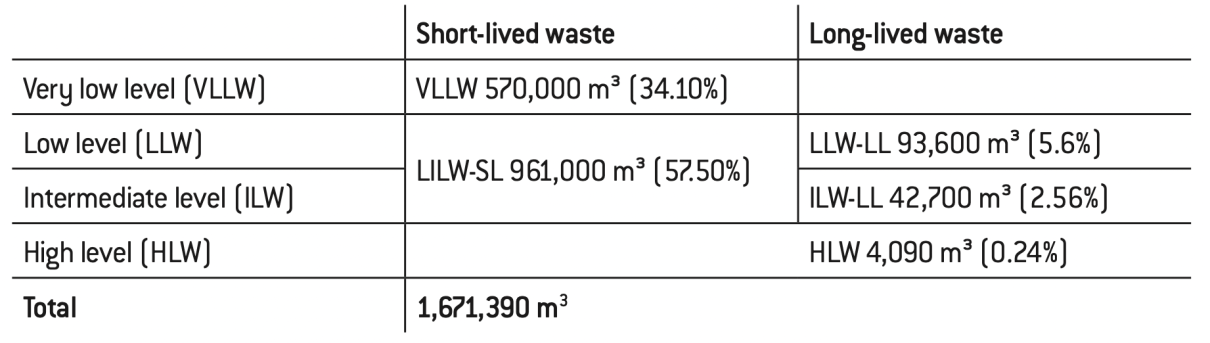
Source :
Andra inventory, 2021.
See Andra, “National Inventory of Radioactive Materials and Waste”, 2021, p. 15.
Gains of about 10% are seen with plutonium mono-recycling and just over 20% can be achieved if reprocessed uranium is also recycled; by comparison, resources are multiplied by 100 with fast neutron reactors.
See Andra inventory, 2021.
When resources like coal and rare earths are mined, radioactive materials naturally present in the Earth’s crust are extracted at the same time.
A word on the toxicity of uranium and plutonium: the level of radiotoxicity of uranium is low, and dominated by its chemical toxicity, of the same order as that of cadmium (effects on kidneys). Conversely, the chemical toxicity of plutonium is low and dominated by its α radiotoxicity, meaning it can be handled by workers with no significant difficulty (negligible risk of external irradiation) as long as it is not ingested or, importantly and imperatively, that dust is not inhaled. Lastly, it should be noted that plutonium is nonetheless much less radiotoxic than the other radioelements found in nature such as polonium 210 (a poison with a sad reputation). The main danger relates to the risk of proliferation (see Henri Métivier, Plutonium. Mythes et réalités, EDP Sciences, 2010).
• Very low-level waste (VLLW) comes primarily from the dismantlement of nuclear power plants and equipment used in the fuel cycle. It is usually in the form of inert waste (concrete, rubble, soil…). France is the only country that considers this waste radioactive without distinction, even though radioactivity is negligible for much of it.
• Short-lived low-level and intermediate-level waste (LILW-SL) mostly comes from fuel cycle operations (treatment of liquid effluents, filtration of gas effluents) and maintenance (clothing, tools, gloves, filters). These objects contain small traces of contamination by radioactive products from the fuel and the materials present in the vessel that were activated by the neutrons in the chain reaction. They are limited in quantity from the start, and of course decrease over time.
• High-level waste (HLW) makes up 95% of radioactivity and is the most hazardous waste. It comes directly from spent fuel and, at 4,000 m3, only constitutes 0.2% of total volumes (the volume of a cube with 16m sides for several decades of electricity generation).
• Intermediate-level,long-livedwaste(ILW-LL)comesfromtheactivation of the structural materials of fuel assemblies (cladding, hulls and end-caps) and the internal structures of the reactor vessel (control rods) as well as effluents associated with spent fuel reprocessing. This explains the intermediate-level classification and the need to ensure protection from it over the long term.
• Low-level, long-lived waste (LLW-LL) is an intermediate category between the 50,000 m3 of HLW and ILW-LL, the most sensitive waste, and the 1.5 million m3 of VLLW and LILW-SL that is less dangerous, particularly over the medium and long terms. It notably comes from the graphite “sleeves” in first-generation natural uranium-graphite-gas reactors (UNGG); these graphite sleeves moderated (slowed down) the neutrons in reactors that used natural uranium, which have now been decommissioned and are in the process of being dismantled.
Some 60% of all the nuclear waste produced in France in recent decades is directly related to the nuclear power industry, the rest being produced by research (much of which is devoted to nuclear power), defence, industry excluding nuclear power, and the medical sector17. VLLW and LILW-SL make up more than 90% of a total volume of 1.67 million m3 but account for a very small share of radioactivity, contrary to HLW.
b) Materials or waste: spent fuel, depleted uranium and reprocessed uranium. How much is recycled?
Nuclear materials are substances for which a subsequent use is planned or envisaged, in some cases after processing, whereas radioactive waste refers to radioactive substances for which no subsequent use can be planned or envisaged based on current knowledge. The distinction made between materials and waste is thus determined, in large part, by the energy and nuclear strategy of the country in which they are located.
As of today, to produce 400 TWh with light water reactors in France, the uranium oxide fuel assemblies require about 1,000t of uranium with enrichment in uranium 235 of 4%. Preparing these 1,000t requires 8,000t of natural uranium (which in turn requires the extraction of 8 million tonnes of ore with natural uranium content of 0.1%). This explains why 7,000t of depleted uranium (low in isotope 235) is produced every year.
At the back end, this leaves 1,000t of spent fuel, including 940t of residual uranium (uranium 235 content of just under 1%), 11t of transuranic elements, of which around 10 tonnes of plutonium and 1 tonne of minor actinides (americium, neptunium, curium), as well as 49t of fission products (iodine, caesium, technetium, strontium…). What remains of the 1,000t of uranium are 950t of uranium and plutonium, or 95% of the original fuel that was not used in this initial combustion and holds considerable energy potential if taken together with the 7,000t of depleted uranium resulting from ore enrichment. It was this observation that inspired France’s long-term strategy, defined in the 1980s and followed until recently, to reprocess spent fuel in order to separate the uranium and plutonium and make it possible to recycle these materials, primarily plutonium and depleted uranium.
The long-term goal is the industrial deployment of fast neutron reactor technology to make it possible to reuse the plutonium and depleted uranium (resulting from uranium enrichment for PWR technologies), as well as the reprocessed uranium. In the meantime, an intermediate step has been taken in recent years that involves reprocessing spent uranium oxide (UOX) fuel and recycling the plutonium resulting from reprocessing in some 20 of the 900 MW reactors in France through mixed oxide (MOX) fuel, a blend with about 92% depleted uranium and 8% plutonium. It is “mono-recycling” in light water nuclear power plants that every year produces spent MOX fuel that awaits reprocessing, currently representing about 2,300t of heavy metal. This initial recycling makes it possible to save natural uranium resources and reuse the plutonium from spent UOX fuel. However, the full energy potential contained in these materials – plutonium (which could be recovered by reprocessing the spent MOX fuel) and depleted uranium – can only be fully used in generation IV fast neutron reactor technologies18. The latter are thus necessary to effectively “close the cycle”, i.e. to use all of the original uranium resource. Later on, we will discuss the potential for technological innovation in the nuclear energy field as well as the strategic choices a country like France must make about the fate of these materials.
If a decision was made to stop reprocessing-recycling these materials, then the spent fuel sitting in stockpiles or in use would have to be added to total waste; in 2019, this would represent about 15,000t of UOX and 2,500t of MOX, not counting the depleted uranium and reprocessed uranium stockpiles, which stood at close to 350,000t19. The first category, spent fuel, is classified as HLW, whereas uranium stockpiles are put together with mining waste, characterised by a very low level of dangerousness.
c) A reminder of some orders of magnitude of waste quantities, especially the most sensitive kinds (HLW and ILW-LL)
Today’s light water reactors require about 8,000t of natural uranium to produce 400 TWh a year, which is three-quarters of the electricity consumed in France. From these 8,000t, 1,000t of enriched uranium is produced for use as fuel in the nuclear reactor cores, which in turn, via controlled fission chain reactions, will produce the energy needed to heat the water in the reactor, with that heat then being transformed into mechanical energy then electrical energy. Of the 1,000t, just 60t is consumed, i.e. undergoes fission or is transformed by neutron capture, and it produces 49t of fission products and around ten tonnes of actinides, mostly made up of about 10t of plutonium and just over 1t of minor actinides (americium, neptunium and curium).
Nearly 85% of fission products are stable or stabilise within a few years (“very short-lived”). This leaves about 7t that are active, just under half (3t) for less than 30 years, meaning they are short-lived (caesium, strontium…), with over half (about 4t) being long-lived.
If we look only at flows in a steady state, without taking into consideration fuel stockpiles that will remain when we cease to use nuclear technology, and factoring in France’s reprocessing-recycling policy mentioned above, which aims, via the use of MOX fuels in existing reactors, and subsequently via the deployment of fast neutron reactors, to make use of the uranium and plutonium in spent fuel with the depleted uranium from the enrichment phase (closed-cycle nuclear strategy), then the only HLW containing long- lived products left are fission products and minor actinides. This represents less than 10t/year if we exclude the materials that are no longer significantly radioactive, and 50t/year including all fission products. This ultimate waste is potentially hazardous, but exists in extremely small quantities, between 0.2 and 1g/person/year, relative to the total amount of hazardous toxic waste humans produce.
If we add in ILW-LL (and LLW-LL), the totals are below 10g/person/year. All waste taken together, including very low-level and short-lived waste (not counting mining waste or similar), adds up to 1 to 2kg/person/year, whereas production of the most toxic hazardous industrial waste approaches 100 to 200 kg/person/year.
This reflects the effects of the very dense and concentrated dimension of nuclear energy. It is the slight loss of mass of the 60t consumed (out of the 1,000t of fuel) as it is transformed into fission products and other actinides (loss of mass of about 50kg) that becomes the massive generation of kinetic energy of new particles via Einstein’s ΔE=Δmc2 equation, producing 400 TWh.
Replacing the 1,000t of nuclear fuel to instead generate 400 TWh with coal would require 150 million tonnes of coal, resulting in the production of 27 million tonnes of solid waste (500kg/person/year) and about 350 million tonnes of CO2 (roughly France’s total CO2 emissions today), not to mention local air pollutants (NOx, SO2, particulates) or the 800t of natural uranium accompanying the extraction of 150 million tonnes of coal… A comparison with other electricity-generating technologies, such as wind and solar power, and the networks and storage they require (batteries, etc.), would be useful, and would also help illustrate the consequences of relying on these technologies that do not emit CO2 (unlike coal or gas) but are much less compact and dense than nuclear, meaning they require considerable amounts of materials, from mine resources including rare earths20 through to the discharges and effluents resulting from the chemical processes involved in manufacturing the main components of solar panels or batteries, and thus waste with an indefinite lifespan if it is chemically stable.
If, by contrast, no effort is made to reprocess or recycle materials with a high energy content, particularly the plutonium in spent fuel (open-cycle nuclear strategy), then that spent fuel must be considered as waste. Countries that have so far opted for the open-cycle strategy include Sweden, Finland and the United States. In this case, long-lived and high-level waste would total 1,000t/year, which works out to about 15g/person/year, as opposed to 1g/person with only fission products and minor actinides in the steady- state closed-cycle strategy. Within this gap, it is mostly the 940t of uranium that make a difference in mass terms, knowing that its level of activity is durable but low since half-lives are 4.5 billion years for uranium 238 and 0.7 billion years for uranium 235. The problem is compounded mainly by the presence of the 10t of plutonium, as plutonium 239 has a much shorter half-life of 24,000 years, and its descendants include americium 241, which has an even shorter half-life of 432 years, and thus a more intense level of activity. If we count only these 10t in addition to the 50t of fission products and minor actinides, we would only be 20% above the 1g per person with the closed cycle. In both cases, the orders of magnitude in mass terms, and volume terms as well, remain very low relative to the other categories of waste produced by humanity21.
These observations illustrate the need to think in specific terms:
– The quantities and dangerousness of waste produced by an industry like nuclear power will naturally depend on the recycling strategy adopted, which is in turn dependent on the industrial technologies mastered (reactors and fuel cycle), knowing that some of the most hazardous waste (at least potentially) can also be the most useful and can disappear via that use: in nuclear physics, it can be eliminated through recycling via the transmutation of materials (this is what happens to plutonium in fast neutron reactors). Energy resources thus increase while waste decreases;
– We must ask ourselves not only about flows of materials and waste in more or less steady-state operations, but also about the waste stockpiles that will be left once a technology or industry is no longer active, which will necessarily happen sooner or later. What will be done with the spent fuel from reactors if nuclear is quickly phased out over the coming decades or in 200 or 300 years, after we have been through several fleets of fast reactors and before we switch over to a new energy production technology that is not yet known today? This notably implies that the need to manage waste that contains limited quantities of plutonium is an issue that may arise with closed cycles as well, though that strategy paves the way for controlling and reducing the quantity of hazardous waste in ways that will be very useful over the long term;
– Lastly, it is important to discuss the potential dangers for health and the environment of these radioactive materials and the different categories of waste before weighing solutions to protect ourselves from them.
The potential dangers associated with radioactive waste (activity, radiotoxicity): some points of comparison with toxic industrial waste
To read more on this topic, see Maurice Tubiana (ed.), Radiologie. Radiothérapie et radioprotection. Bases fondamentales, Hermann, 2008.
Trying to assess potential danger is complex since the level of danger depends on a variety of factors: the intrinsic characteristics of each of the products in question (radioactive period, energy and type of radiation emitted), but also how they can circulate through our environment and enter into contact with the human body and its different organs. Time is an especially important factor to take into account given that radioactivity decreases with time. Whereas very toxic industrial waste is often stable, the hazards associated with nuclear waste change considerably over time, depending on the half- life of the different radionuclides. It is also important to consider how long these toxic products remain in the human body, which is referred to as their biological half-life. Ensuring adequate protection from these potential dangers requires taking all these factors into account.
a) The possible health consequences of radioactivity for humans: from becquerels to sieverts22
Radioactive materials contain unstable atomic nuclei that are transformed when they disintegrate, emitting material radiations, helium nuclei or electrons – referred to as α or β radioactivity – or electromagnetic radiations, very high-frequency, very short wavelength photons, known as γ radioactivity. These radiations then interact with the material they traverse, transferring energy to it mainly via ionisation and atom excitation. When they reach living beings, these radiations lose their energy in all tissues traversed, and may randomly affect the cell nuclei of those organisms and the DNA of those cells via direct shock or, more generally, via the creation of free radicals (through radiolysis of the water around the DNA) that will chemically attack the DNA. Few other molecular targets inside the cells, with the exception of the enzymes involved in DNA repair, have the same consequence for cell survival or mutagenesis. This mechanism of toxicity is not unique to ionising radiation. The effects can be observed following aggressions of various types, chemical or thermal, or by ultraviolet light, and physiological, during cellular respiration (aggressions that can notably come from products arising from industrial or chemical waste from other means of energy production, knowing that the chemical toxicity of the latter can also use other routes such as disruption of the enzymatic environment).
Of course the severity of the effects of this radiation will depend on the dose received. The latter is determined by three key parameters: the activity of the radionuclide, the energy of its radiations (higher for α, lower for β and γ), and the impact on humans (sieverts) which is a function of the nature of radiation (α-rays are more radiotoxic) and, most importantly, of the sensitivity of the organ (taking into account how long the radionuclides are retained, i.e. their biological half-life or period).
The activity of the radionuclide that is the source of the radiation, i.e. the number of rays emitted per second or, in other words, the number of disintegrations per second, measured in becquerels (Bq) regardless of the radiation emitted, depends on the quantity of radioactive material and its mass activity in becquerels per gram (Bq/g).
At this stage, three comments of a technical nature can help explain the sometimes high and very different levels of mass activity (see table 2):
– The unit considered – the becquerel per gram – often yields high numbers by construction since 1g of material contains a very large number of atoms. The order of magnitude is comparable to that of Avogadro’s number (the number of atoms in one mole, i.e. 6.02 1023) – close to 1022 or 1023 depending on the mass number of the atom of the material in question, and thus a high number of disintegrations if an even weakly radioactive material has been purified and concentrated. It will be easier to confine if volumes are low, and the mass activity will of course be greater, the opposite of the result that would be obtained with a dilution strategy;
– The period of the radionuclide is the key determinant of activity. The longer the half-life or period of the radionuclide, the lower its mass activity: uranium 238 has a half-life of 4.5 billion years (approximately the age of planet Earth) and its mass activity is just 12,434 Bq/g, definitely very low compared with a mass activity of 4.6 million billion Bq/g for iodine 131, a fission product with a very short half-life of eight days, or the mass activity of 3,200 billion Bq/g for caesium 137, a fission product with a half-life of 31 years (the very wide range of orders of magnitude of mass activity is the result of the combination of powers of close to 1023 of the number of atoms in 1g and radioactive periods that indicate the time it takes for half the atoms in that gram to disintegrate, bearing in mind that the number of seconds in a year is close to 3.2 106);
– The third and final important comment to make relates to the exponential character of radioactive decay: iodine 131, a fission product with a very short half-life and present in the fuel used during a reactor shutdown, appears to be extremely active, but not for long, as its half-life is only eight days.
This means that its mass activity will be halved every eight days and reduced by a factor of 1,000 every 80 days (210 ≈ 1 024) and by 1030 in 800 days (meaning it will be negligible after one to two years). It should also be noted that in the event of a significant iodine leak following a serious accident, iodine tablets should be taken prior to contact with the radioactive source so that the body will not take up this dangerous element in the thyroid within the first weeks. In other words, properly assessing effective risks requires understanding all the main parameters that determine them, including protections that are simple and available, and should be on hand in case of potential danger.
As regards the energy contained in the radiation, the dose absorbed by the human body or non-human living organism depends on the energy communicated by the radiations to the irradiated tissue. It is measured in grays, the term for the energy of ionising radiation that transfers one joule of energy to a homogeneous environment with a mass of 1 kg. The absorbed dose is linked to the quantity of the radionuclide and its mass activity, also taking into account the energy characteristics of the radiation emitted (see table 2). Short-lived fission products like caesium (half-life of around 30 years) have considerable γ-ray energy. They will thus have an effect in the first decades but disappear fairly quickly on a scale of two or three centuries. Long-lived fission products, technetium 99, iodine 129, caesium 135, are mostly β emitters (they rarely emit γ-rays) of less than 200 or 300 keV, moderate energy levels. They can also emit X-rays via the “braking radiation” of these β electrons. And long-lived actinides, including americium 241 (432 years) and plutonium 239 (24,000 years), emit high- energy α-rays of around 5 MeV, but almost no γ-rays (except americium: low-energy γ-rays of 60 keV).
Table 2: Mass activity and dose factors for a few radionuclides
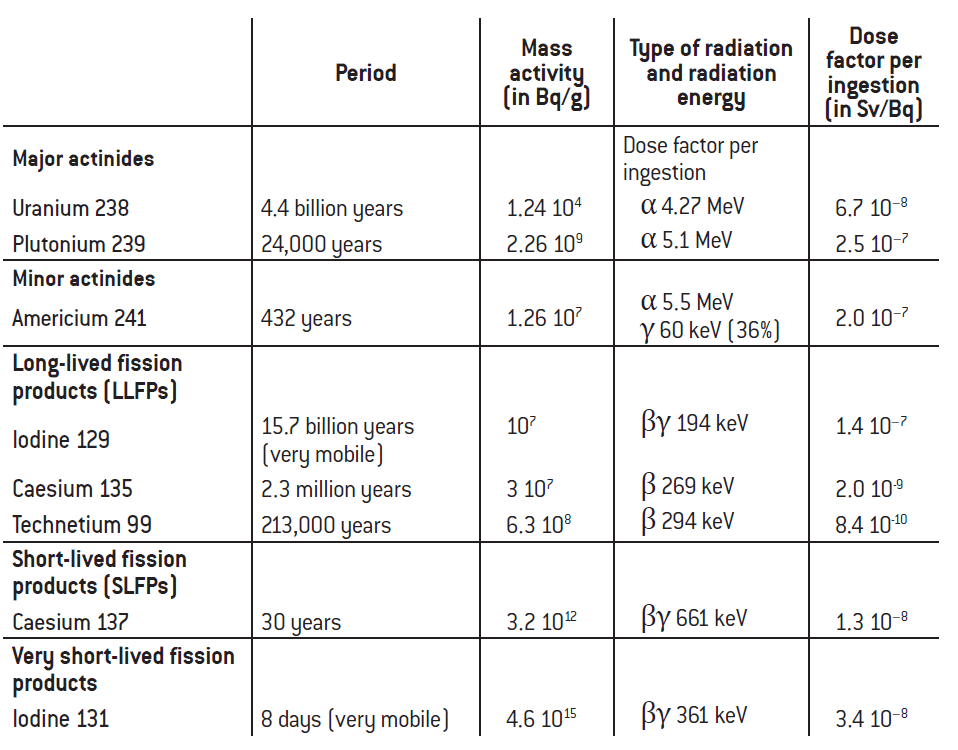
Source :
Robert Dautray, L’Énergie nucléaire civile dans le cadre temporel des changements climatiques. Rapport à l’Académie des sciences, Tec & Doc, 2001, p. 252; Jean Lefèvre (dir.), Les Déchets nucléaires, Eyrolles, 1986; Bernard Bonin (dir.), Les Déchets nucléaires: état des lieux et perspectives, EDP Sciences, 2011, p. 33; radioactivité.com; Wikipédia; for dose factors, see the Official Journal of 2003.
To read more about these radiation protection issues, see, among others, Bernard Bonin (ed.), Les Déchets nucléaires : état des lieux et perspectives, EDP Sciences, 2011.
The impact on humans (measured in sieverts) is a function of the nature of the radiation (α- versus β- and γ-rays) and the sensitivity of the organs affected. Different notions have been constructed to take account of these two factors and measure the potential noxiousness of radionuclides23.
For the same absorbed dose, the biological effects will vary depending on the nature of the radiation. It is to take this factor into account that the notion of “equivalent dose”, measured in sieverts, is applied. It introduces a coefficient that reflects the greater toxicity of α relative to γ and β, by a factor of 20 for the same energy measured in grays (in other words 10 Sv = 10 Gy in the case of γ-rays and 10 Sv = 0.5 Gy x 20 in the case of α-rays).
To reflect the sensitivity of the different human tissues, the concept of “effective dose” is used, which, by applying a weighting factor to tissues, takes into account the relative contribution of each tissue to the overall stochastic risk in the event that the entire body is irradiated in a homogeneous manner. It too is measured in sieverts.
In cases of ingestion or inhalation (“internal contamination”), the “dose factor” measures the noxiousness of a radionuclide. The factor is specific to each radionuclide, and it is evaluated using a model that describes the path of the radionuclide as it travels through the different compartments of the body. It takes into account that not all radionuclides have the same radiation, and that their path of access in the body is different. The table of dose factors published by the ICRP is regularly updated as new knowledge becomes available.
Jean-Marc Cavedon, La radioactivité est-elle réellement dangereuse ?, Le Pommier, 2014, p. 46.
To read more about the issue of low doses, see Bernard Le Guen and Roland Masse, “Effets des faibles doses des rayonnements ionisants”, EMC Toxicologie-Pathologie professionnelle, 16-510-A-10, 17 July 2007, and Roland Masse, “Effets sanitaires des faibles doses de rayonnements ionisants : origine et actualité d’une controverse”, Pollution atmosphérique, Special “Énergie-Santé” Issue, June 2014, p. 27-38.
A few basic facts about radioactivity and its health impacts Measuring health impacts and orders of magnitude associated with radioactivity • Activity of a radionuclide is the number of disintegrations its nuclei undergo per unit of time, 1 Bq = one disintegration per second (regardless of the radiation emitted, α, βorγ). • Irradiation dose (or “absorbed dose”) measures the energy deposited by radiations in a medium per unit of mass of that medium: 1 Gy = 1 joule/kilogram. • The same quantity of energy deposited by a radiation will not produce the same biological effects, depending on the nature of the radiation (α, β, γ, X) and that of the organ irradiated. The measurement used to quantify the biological effects (noxiousness) of radiation affecting living tissue is the sievert (Sv). • α-rays, which do the most damage to tissues, can easily be stopped by a sheet of paper or the outermost layer of the skin. They thus only pose a problem in cases of internal contamination through ingestion or especially inhalation. External irradiation is primarily linked to high-penetration γ-rays, and lower-energy X-rays. β-rays are low-penetrating and less harmful than α-rays in the event of internal contamination. • Natural radioactivity in France is close to 2.4 mSv/year on average, and depending on the region, natural exposure ranges between 1.5 and 6 mSv/year. Natural exposure can be as high as 50 mSv/year in parts of the Kerala region, in southern India, or 35 mSv/year in Espírito Santo in Brazil. Of the 2.4 mSv/year of natural exposure in France, 0.4 mSv/year comes from cosmic rays (1.7 mSv/year at 4,000 metres of altitude), 0.7 mSv/year from telluric radiation emitted by radioactive elements (0.4 mSv by external irradiation, 0.3 mSv by ingestion – potassium 40, lead, traces of polonium) and 1.3 mSv/year by the inhalation of radon (also of telluric origin). • Additional exposure in France stems primarily from medical diagnostic activities for about 1 mSv (a body scanner between 4 and 10 mSv), with technological and industrial activities accounting for a small share (0.1 mSv) and the nuclear power industry for a negligible percentage (less than 0.1% of natural radiation exposure). • Deterministic effects, which appear quickly and with certainty above a certain dose (threshold). The farther above the threshold the dose, the more serious the damages (due to the destruction of cells of the same tissue). The lethal dose is close to 10 Sv and the LD50 (50% likelihood of death) is near 5 Sv. No effects are observed below 0.5 Gy, while a general and reversible reaction is seen at between 0.5 and 2 Gy and serious illnesses at 2 Sv or above. • Probabilistic effects, which translate into uncertain damages that may appear after a period of time, shorter or longer depending on the organ affected. This damage stems more from cells mutating than from cells being destroyed. It is the probability of damage appearing (cancer, leukaemia), not its severity, that increases with the dose received. Within a given human population that is exposed, no discernible cancer risk is seen below 100 mSv. A linear relationship has been observed above that, with a 0.5% increase in the probability of developing a fatal cancer with every 100 mSv (+25% probability for 5 Sv*). The probabilistic effect of low doses (below 100 mSv) is debated, knowing that pregnant women and young children could present sensitivity at lower doses**. • The radiation protection thresholds defined by the ICRP include a very significant safety margin, with thresholds set at 20 mSv/year for nuclear industry workers and at 1 mSv/year for the rest of the population. The limits set by French nuclear safety authority ASN in terms of risks around geological disposal sites (for the few persons living nearby) over 100,000 or 1 million years are even lower, at 0.25 mSv. |
Taking into account the specific radiosensitivity of the organ or tissue irradiated depending on the radionuclide, as well as the mode of exposure (internal contamination, ingestion or inhalation vs. external irradiation), the potential radiotoxicity of a radionuclide can be defined. It characterises the health risk posed to a human body by the ingestion or inhalation of the radionuclide in entirety, also measured in sieverts (here, the effective dose taking into account weighting factors for the tissues affected). It can be calculated as the product of the radioactivity of the radionuclide in becquerels multiplied by the unit radiotoxicity of that radionuclide inhaled or ingested and based on the age of the subject (the “dose factor” in sieverts/ becquerels)24.
We can thus observe that once incorporated into the organism that ingested or inhaled them, the actinides plutonium, americium and curium have an impact measured in sieverts that can be 100 to 10,000 greater than that of LLFPs with the same activity. This effect is due to the combined action of the energy from the α emissions, their quality factor, and the persistence of the actinides, over their life, at the sites where they are retained: lungs (after inhalation), bones and liver. That being said, one important factor limits their impact after migration in the environment: their very low mobility and very low alimentary tract transfer factor (5 for 10,000 in adults25). This considerably limits the impact via the digestive tract, as during transit, the α-rays only reach a few cellular layers of the digestive tube, but not the cells that renew it. Due to the very short range of α particles in the air, the impact on humans can only be caused by internal contamination by the actinides. Like environmental pollutants, heavy metals, arsenic, dioxins and cyanide, actinides can only be toxic if ingested or inhaled.
The concept of potential radiotoxicity must thus be discussed with caution, since by definition, it assumes that the entire product is ingested (or inhaled), without considering its mobility or solubility in the environment, or the effective protections that can be put into place to prevent the inhalation or ingestion of radionuclides, as can be done with any toxic industrial product. The fact is that most of these actinides are effectively not very mobile or soluble, and protections, if well thought-out, including over long timescales, will stop the radionuclides from entering the human body, thus substantially reducing risk.
Moreover, external irradiation by high-energy β particles (and the related braking radiation (X) and, especially, more penetrating γ-rays (protection against which is particularly important in the first decades and centuries for short-lived fission products), is a pathway that is specific to radioactivity: we take a risk when we approach a β-γ radioactive material. This danger can be avoided by using screens that stop these rays; the safeguards are simple, and this radiation can be measured with great accuracy (with a simple Geiger counter). Yet this pathway may seem mysterious, similar to thinking about the air being polluted with odourless and invisible molecules such as CO2, if we do not have access to these instruments to measure them and to these protections.
b) Evolution over time of the radioactivity and potential radiotoxicity of spent fuel and high-level waste
Evolution of activity (becquerels)
Graphs 1 and 2 below compare the evolution of activity of HLW glasses and spent fuel to the activity of the natural uranium ore required to fabricate that fuel (the latter activity, measured by tonne of heavy metal in the enriched fuel, thus corresponds to 8t of natural uranium with its descendants, which is about 100 times the activity of a tonne of natural uranium without its descendants).
Graph 1: Decay of the sum of actinides and the sum of fission products over time after reprocessing (activity equivalent to 1t of heavy metal in the glasses)
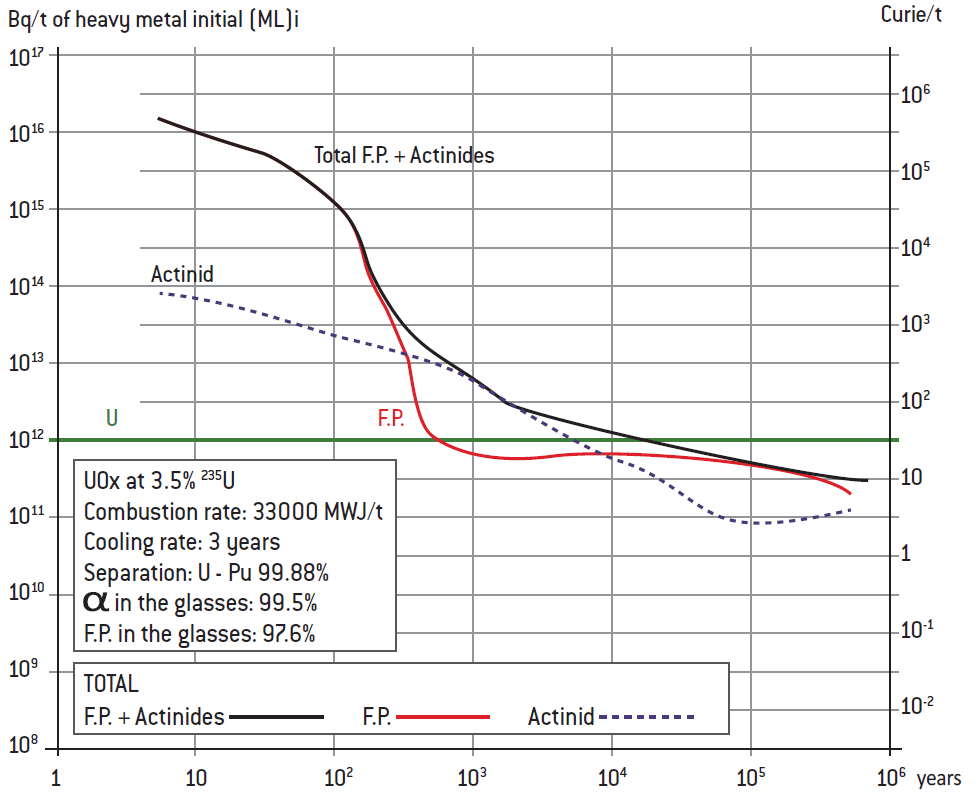
Source :
Robert Dautray, L’Énergie nucléaire civile dans le cadre temporel des changements climatiques. Report submitted to the French Academy of Sciences, Tec & Doc, 2001.
* Equivalent activity (including descendants) for the quantity of ore necessary to fabricate a tonne of heavy metal.
Graph 2: Decay of the radioactivity of a fuel element before reprocessing
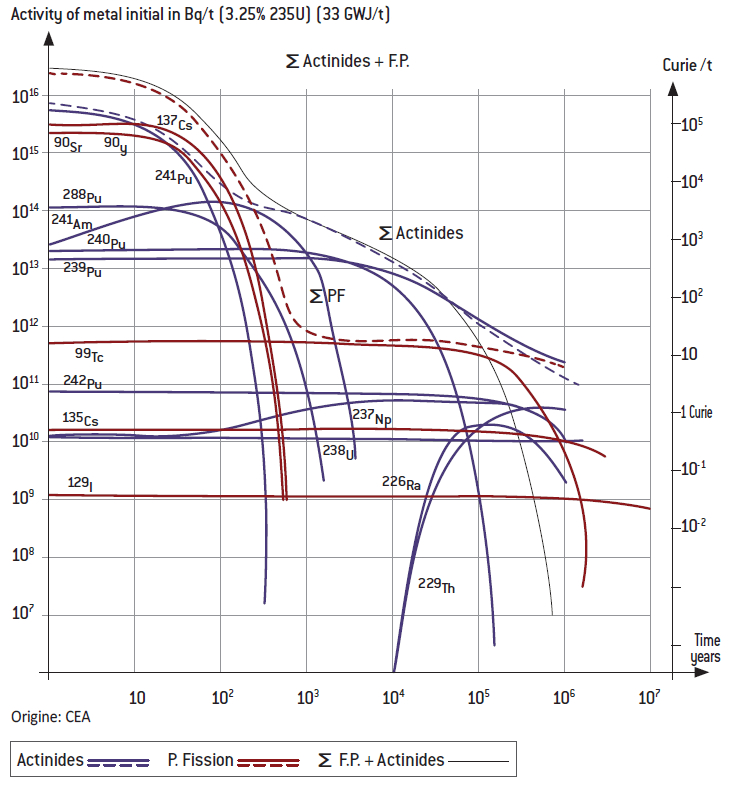
Source :
Robert Dautray, L’Énergie nucléaire civile dans le cadre temporel des changements climatiques. Report submitted to the French Academy of Sciences, Tec & Doc, 2001.
See Christian Ngô and Alain Régent, op. cit., p. 37.
Fission products decay rapidly, with their level of activity matching that of uranium ore within a few hundred years (about 500); glasses do the same in 10,000 years and spent fuel (with plutonium) in just over 100,000 years.
Within spent fuel, fission products that are short-lived and emit γ-rays (such as caesium with its half-life of 30 years) and high-energy β emitters (like strontium with the same half-life) account for the bulk of activity in the first 500 years. Next come americium 241 (period of 432 years), up to 2,000 years, and then plutonium 239 (24,000 years), from 2,000 to 100,000 years. Both are α emitters. Technetium 99 (213,000 years), a fission product and low-energy β emitter, dominates between 100,000 and 1 million years, beyond which time the other α actinides with even longer half-lives such as neptunium 237 become dominant.
In the glasses, a similar evolution is observed without the presence of plutonium (and its descendants). Americium thus dominates up until a few tens of thousands of years, before technetium 99 takes over.
Activity immediately after leaving the reactor is very high, and yet already well below the number of fission reactions inside a reactor in operation. The level upon leaving the reactor is already a few thousand times below the number of reactions that occur per second when the fuel is in the reactor and producing energy. The considerable activity inside the reactor in operation is confined by three successive screens: the fuel cladding, the steel vessel and the concrete in the reactor building26. Though the activity level remains very high upon leaving the reactor, it decreases by a factor of 50 to 100 within the first ten years due to the very rapid decay of very short-lived fission products like iodine 131 (eight days). The level then decreases rapidly again, by a factor of 1,000, in 300-500 years, due to the decay of short-lived, γ-emitting fission products such as caesium (period of 30 years), after which the decay rate slows considerably, following a downward plateau where the loss is barely above a factor of 10 between 300 years and 1 million years.
The potential danger associated with external irradiation has been decreasing sharply during the first 300-500 years. From that date (300 years) and up until 100,000 years, the activity in HLW glass is dominated by that of actinides, which emit dangerous α-rays if ingested or inhaled, but are heavier and less mobile than fission products, and thus easier to confine in a defined space (which at the same time makes it possible to ensure protection from the related rare residual γ-rays, particularly for americium). The residual danger of external irradiation beyond 300 years probably stems, during the long downward plateau, from the braking radiation (X-rays) caused by the slowing of the β particles in long-lived fission products like technetium 99, on the one hand, and on the other to the contribution of other long-lived radioactive elements. It is best to continue to avoid staying near this waste for any extended period without protection, given the concentration of radioelements.
See Louis Patarin (ed.), Le Cycle du combustible nucléaire, EDP Sciences, 2002, p. 160.
Robert Dautray offers a similar calculation: “If we are looking at the ratio of radioactivity added to that of the Earth’s crust, the ratio of total radioactivity over 40 years of operation of the global nuclear power plant fleet is of the order of 10–7 right after fuel leaves the reactor and then decreases to 10–11 in a very short period of time relative to a geological timescale, then below zero over these timescales, since these very long-lived bodies are destroyed and replaced by others, most of which have a much shorter lifetime”. (Robert Dautray, L’Énergie nucléaire civile dans le cadre temporel des changements climatiques. Report submitted to the French Academy of Sciences, Tec & Doc, 2001, p. 52).
The radioactivity nuclear waste adds to that of the Earth’s crust
The activity of a glass package, at the time it is constituted, is close to 16,000 TBq*. This means that for a nuclear power fleet producing 400 TWh a year over 50 years, activity is close to 5 1020 Bq a few years after the packages leave the reactor (it takes two glass packages for the waste associated with 1 TWh). The volume of the Earth’s crust under France is close to 2 1016 m3. This corresponds to the surface area of France – around 500,000 km2 – multiplied by the 40 km average depth of the Earth’s crust. With activity associated with uranium and thorium, as well as their descendants at equilibrium, of about 1,000 Bq/kg, the total natural activity of the Earth’s crust under French soil is in the neighbourhood of 6 1022 Bq. The initial activity of the glasses thus corresponds, during the first decades, to 1% of the activity in the subsoil. At the scale of a few centuries, this activity will decrease by a factor of around 104 due to the rapid radioactive decay of short-lived fission products. At a 500-year horizon, the level of activity corresponds to that of 4cm of the French subsoil, a good illustration of how negligible the radioactivity of waste is over the long term relative to natural radioactivity. We are actually quite far from the image of Promethean activity. While the significant danger of this waste stems from its very high concentration in a very small volume, this very same quality will make it possible to design simple confinement solutions. It should also be noted that over the very long term, beyond a million years, radioactivity on earth will have diminished since very long-lived materials (uranium) will have been destroyed in reactors and replaced by others with a shorter lifetime**. |
Evolution of potential radiotoxicity through ingestion
We often use the notion of potential radiotoxicity: this refers to the hypothetical dose a set of individuals would receive after ingesting the totality of the radioactive material considered (see graph 3, in sieverts per tonne of spent fuel). After the initial decades when the γ -rays from very short-lived and short-lived fission products dominate, it is primarily plutonium that dominates over the long term and, if it is recycled, then the minor actinides are the source of the most radiotoxicity. This domination of plutonium in terms of radiotoxicity explains the interest in transmuting it to eliminate the dangers posed by its ingestion and inhalation (noting, however, that the main benefit of plutonium transmutation relates to the ability to use its energy potential). The possibility of transmuting minor actinides is a secondary area of research aimed at further reducing the radiotoxicity of waste.
It is important to underscore the theoretical dimension of this notion of potential radiotoxicity and to exercise caution in interpreting its consequences. It must be borne in mind first that these heavy atoms are not very mobile or soluble, and second, that they are easy to confine in matrices- packages-geological repositories.
Graph 3: Evolution over time of potential radiotoxicity through ingestion
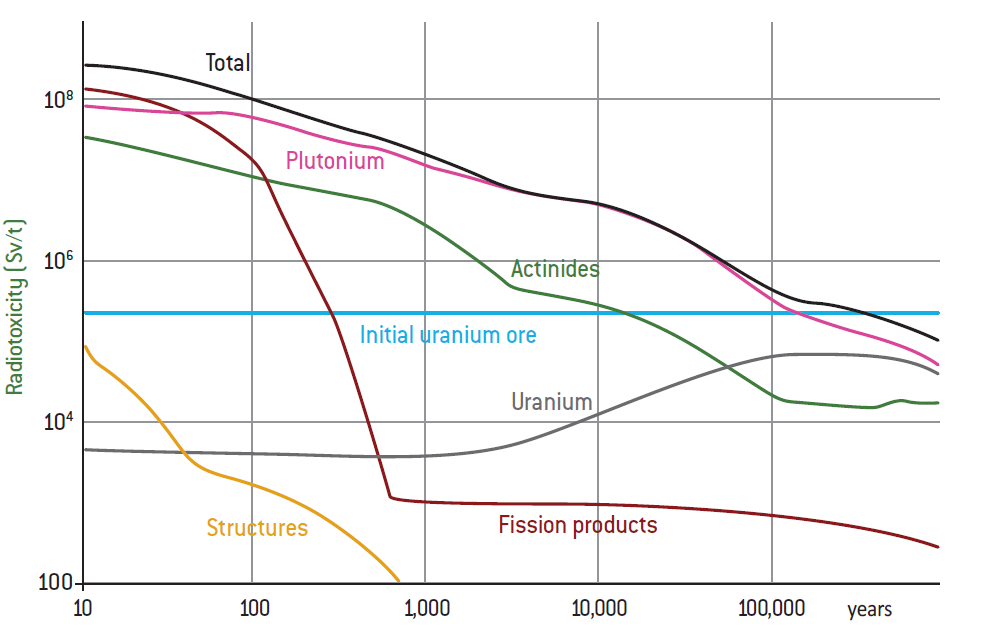
Source :
2005 Andra and CEA dossiers
See also Figure 1.2, Radiotoxicity evolution in time and its components, in “Physics and Safety of Transmutation Systems A Status Report”, OECD, 2006, p. 9.
See Jean Lefèvre (ed.), Les Déchets nucléaires, Eyrolles, 1986, p. 37.
These orders of magnitude are based on the decay curves of the fission products in the glass and on recent analyses in an IRSN report entitled “Méthodologies et critères envisageables pour apprécier la nocivité des matières et déchets radioactifs”, Report N°. PSE-ENV/2018-00048, Health and Environment Division, September 2018).
OPECST, “Effects of heavy metals on the environment and health, Messrs Gérard Miquel and Henri Revol, Senators”, Senate, Information report No. 261 submitted on 5 April 2001, p. 108, 99 and 135.
c) Comments on the potential dangers associated with these different products
Potential dangers linked to external irradiation: a risk that characterises the γ-β of fission products
This risk relates especially to γ-rays, less so to X-rays, and very little to β-rays. Long-lived fission products only present, where caesium 135 and technetium 99 are concerned, low-penetrating β-rays, stopped by a few millimetres of water, a few metres of air, or a sheet of metal, and they are low-energy. Caesium 135, with its half-life of 2.3 million years, thus has a low level of specific activity, essentially involving β-rays.
Technetium 99 has a shorter period of 213,000 years, which explains its weighting in the activity of waste or spent fuel around this period, with essentially β radiation and related X-rays, though the absence of high-energy γ-rays limits its impact. Iodine 129 has high mobility and γ-rays that are a bit higher energy, but its very long period of 15.7 million years significantly reduces its activity, and thus its possible effects (including through external contamination).
This leaves short-lived fission products, caesium 137 and strontium 90, both of which have a half-life of around 30 years. They can be transferred to humans through the alimentary tract. Caesium 137 emits high-energy γ-rays of 600-700 keV, and five years after it leaves the reactor represents nearly27 all γ-rays from fission products . It takes several decimetres or metres of concrete, or a few centimetres of lead, to stop these γ-rays made up of energetic photons. This is why it is indispensable to start by protecting workers and communities from external exposure associated with these materials during the decades and centuries after their removal from the reactor via appropriate screens: matrices-packages-containers-structural elements of storage sites. Their very high concentration and density makes this feasible with no real difficulty.
Calculations based on an IRSN report on the abandonment of a HLW glass package, without any specific protections in place (theoretical assumption of the total absence of protection), suggest some orders of magnitude of the potential danger from external radiation28. A glass package (there are 800 for 400 TWh/year in France) delivers at 1m a dose of about 15 Sv/h at 50 years and 4 mSv/h at 300 years. Caesium 137 is a major determinant of external irradiation over this period, and we see a significant decrease in its activity by three orders of magnitude. Standing 1m away from it is lethal in 1h at 50 years. At 300 years, the dose received in remaining nearby for 1h is equivalent to natural radioactivity. Proximity to it for 365h (1h/d at 1m) would result in receiving a dose of about 1.5 Sv, implying deterministic effects (burns, skin lesions…) and a probability of deadly cancer that increases by about 7% above normal (plus 0.5% per 100 mSv). The impact of the radiation decreases with the square of the distance: at 10 to 100 metres away, levels become comparable to or lower than natural radioactivity. In other words, this external irradiation poses a limited danger after 300 years as long as one maintains enough distance from the package, but it then decreases only very slowly, with a long plateau, losing only one X-rays associated with the delayed β-rays in long-lived fission products (LLFPs) like technetium 99 (and perhaps also the γ-rays of americium). The very high concentration of LLFPs across a few thousand packages, representing very small volumes but still significant danger over time, thus makes it necessary to implement efficient confinement over the long term as well.
The potential dangers of α particles when inhaled or ingested – comparison with toxic industrial waste
α wastes (plutonium, americium) pose dangers when ingested or inhaled, like products found in toxic industrial, household or agricultural waste which are also found in many objects used or handled by humans: heavy metals (mercury, cadmium, lead), arsenic, cyanide (CN), organochlorine compounds (TCDD dioxin, Seveso), organophosphate compounds (C4H10F02P sarin gas, VX, parathion C10H14NO5PS), botulinum toxin (C6760H10447N1743O2010S32), etc.
These products, in significant doses, cause acute toxic effects; in doses that are lower but repeated over time, they can cause chronic toxicity and may also, with the exception of the botulinum toxin, have carcinogenic or mutagenic effects. The LD50 (lethal dose at 50% by ingestion in grams, milligrams or micrograms per kilo) in rats is of course a very insufficient and overly specific indicator of toxicity, though it does help illustrate how the most toxic nuclear waste over the long term (like plutonium) compares to these chemical substances considered as hazardous waste, substances that are used in quantities often several orders of magnitude greater with some released into the air, water or soil.
It is useful to recall, for instance, some of the figures the OPECST report, directed by Gérard Miquel and Henri Revol in 2001, compared to the 10t of plutonium and the tonne of minor actinides produced every year to operate the French nuclear fleet:
– Emissions of heavy metals into the air in France (forecast for 2002): arsenic, 18.5t; cadmium, 14.1t; chrome, 90.8t; mercury, 33.8t; lead, 12.1t;
– Annual consumption in France: cadmium around 1,800t; lead around 273,000t; production of arsenic in France, 10,000-12,000t in 1990;
– Discharge of heavy metals into the water annually: 2.92t of cadmium, 11.79t of arsenic, 33.7t of lead, 341.8kg of mercury, 779t of chrome29.
Table 3: Comparison of the toxicity of several hazardous products
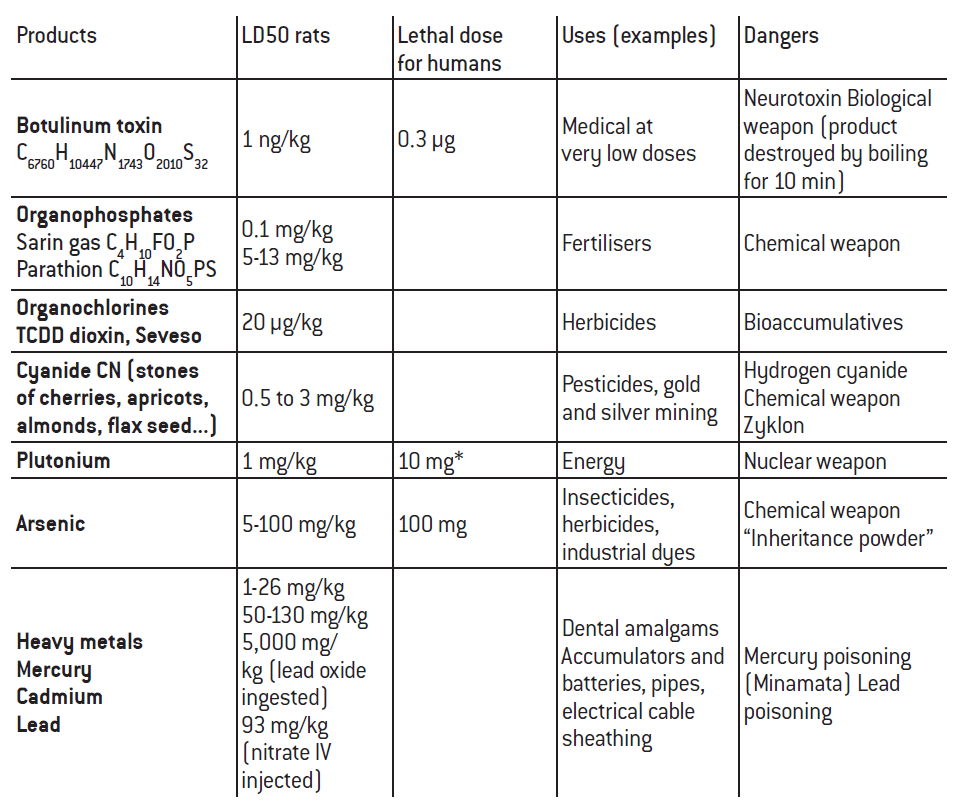
Source :
INRS, IRSN.
* Refers to the “theoretical” dose inhaled (see IRSN, “Radioprotection, radionucléides. Plutonium-239”, fiche ED 4320, June 2014, and Henri Métivier, Plutonium. Mythes et réalités, EDP Sciences, 2010, p. 186). The dose ingested is probably higher given the short “biological half-life” in the gastrointestinal system.
These products often have possible uses, one example of course being plutonium, with its high energy potential, which explains the interest in developing recycling technologies. Another question that arises for many of these products relates to the choice between concentration- confinement versus dilution-diffusion: protection can be ensured either by concentrating this hazardous waste to limit its volumes and facilitate long-term confinement – involving, by construction, doses that, if released without controls, would be very high at the local level – or, on the contrary, by diluting it and accepting its diffusion at low doses (this is the case of discharges from the industrial sector and spreading in the agricultural sector of some products that may be toxic at high doses). The concentration of hazardous products requires paying close attention to the related risks, particularly in the event that the compounds come into contact, potentially reacting to one another and triggering an explosion and/or fire. A similar issue arises with plutonium in spent fuel, with a criticality hazard that must in all cases be controlled.
To study the potential dangers of this HLW when ingested, the IRSN30 looked at the health consequences for an adult and child eating only products from a small 3,000 m2 cultivated plot and a 1 ha body of water contaminated by the abandonment of a HLW package and the dispersion of the totality of its constituents into the plot and body of water. After 1,000 years, the dose is of the order of 1.45 Sv for the child and close to 500 mSv for the adult, implying an increase in the probability of cancer of about 2% for the adult and worrisome consequences for the health of the child. Note that it is a long-lived fission product – once again technetium 99 – that produces 90% of this toxicity, probably because of its greater mobility and transmission within the food chain, while minor actinides, which are less mobile and soluble, account for less than 10%. Moreover, as is clearly stated in the IRSN document, the assumed full lixiviation of the package after one year factored into the calculation is not realistic if we take the glass matrix into account31.
Dispersion in the form of dust in a confined space of all the products in a glass package would be two to three orders of magnitude more dangerous, since the inhalation of actinides into the lungs is extremely toxic. These scenarios corresponding to accidents (in a rather confined atmosphere) are relevant today in seeking to ensure the protection of workers and communities when materials are being handled to be worked, transported or stored, but they are less meaningful if we want to look at the possible health consequences for distant future generations of the abandonment of a glass package.
Taken together, all these elements give us a better idea of the potential long-term dangers of waste, dangers that are comparable to those of other toxic products but are of great importance at the local level due to high geographic concentration in low volumes. Hence the focus on protecting humans and ecosystems by taking advantage of their small quantities and traceability (see Volume 2).
No comments.