Nuclear waste: a comprehensive approach (2)
Solutions to limit effective risksSolutions to limit effective risks for present and future generations
Key takeaways from Part III (regarding the most radioactive and long-lived waste)
The recycling/confinement/storage-disposal solutions used today
Geological disposal in France and the world
The technological advances that could pave the way for sustainable use of uranium resources and the partitioning and transmutation of waste
Summary
Solutions for limiting the risks associated with radioactive waste are based on two principles: reduce waste quantities at the source and prevent radionuclides from reaching humans and spreading into the environment. Nuclear waste is the most dangerous in the first decades after it leaves the reactor, as this is when it emits the most radiation and heat. Ensuring protection against this radiation involves simple steps: place water, concrete or lead between these products and human beings. A variety of solutions for the different categories of waste are currently being implemented in France under the supervision of French nuclear safety authority ASN.
They offer effective protections to people and the environment on a time horizon of two to three centuries.
A very small percentage of this waste requires very long-term solutions to protect future generations from it. Thanks to the extensive scientific research conducted in recent decades in France and internationally, suitable long-term solutions are now available combining long-term storage, transmutation and geological disposal (Cigeo project in France). Of the solutions available, the international community considers geological disposal as the benchmark.
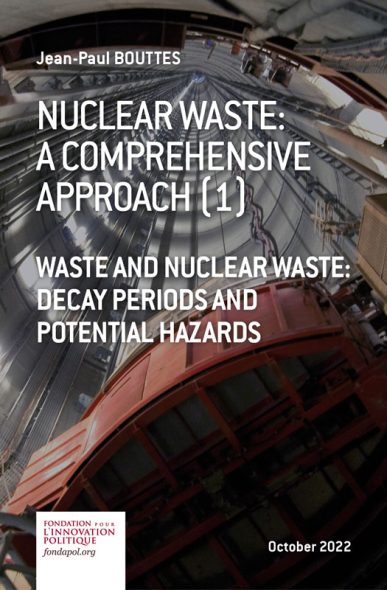
Nuclear waste: a comprehensive approach (1)
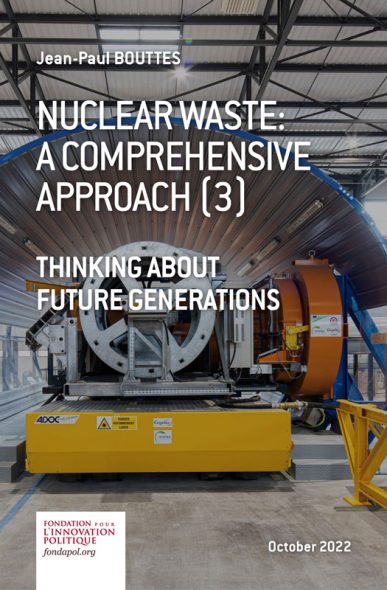
Nuclear waste: a comprehensive approach (3)
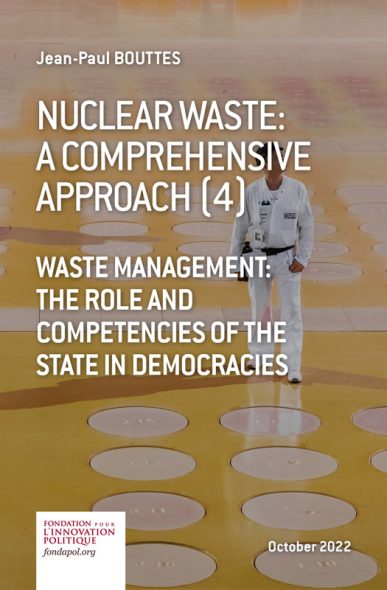
Nuclear waste: a comprehensive approach (4)
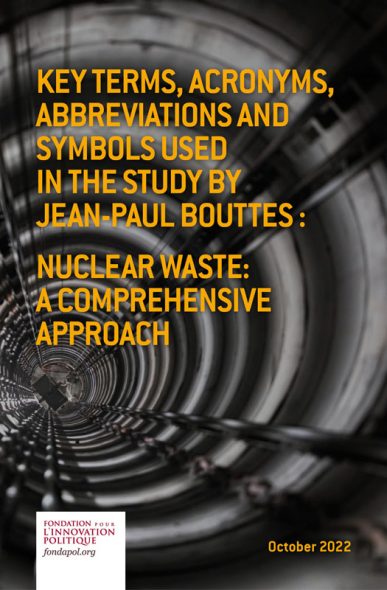
Key terms, acronyms, abbreviations and symbols used in the study by Jean-Paul Bouttes: "Nuclear waste: a comprehensive approach"
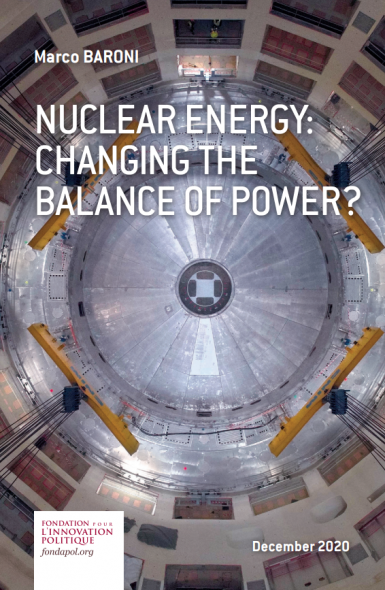
Nuclear energy : changing the balance of power
Solutions to limit effective risks for present and future generations
Key takeaways from Part III (regarding the most radioactive and long-lived waste)
Key data and management of these wastes over a few decades and centuries
High-level long-lived waste comes directly from spent fuel, once that fuel is “combusted” in the core of nuclear reactors. If nuclear is used with an “open fuel cycle” (as is the case in the United States and Finland), meaning no action is taken to reprocess the energy materials not used during this first “combustion” (plutonium and residual uranium, representing 95% of the initial fuel), then the waste is simply spent fuel. With a closed cycle (this being France’s objective), reusable materials (plutonium and uranium) are separated from the “ashes” of this first combustion (fission products and minor actinides), such that the waste that remains is primarily made up of high-level waste, i.e. these fission products and minor actinides, which are placed in glass matrices. In both cases, very small quantities are involved: 50t/year for HLW with a closed cycle, i.e. 1g/person/year (and 1,000t/year for spent fuel with an open cycle approach, i.e. 20g/person/year). This waste is more dangerous when it leaves the reactor and during the first decades that follow as this is when it emits more radiation and heat. Because radioactive decay is very fast during the first three centuries, the risk of external radiation on that time horizon is greatly reduced. Simple solutions are available to ensure protection against this radiation in the first decades and centuries: it suffices to place water, concrete or lead between these products and humans or the environment. This work is made that much easier by the fact that the volumes in question are very low, traced, and rigorously and accurately monitored through measurement instruments (Geiger counters) under the control of French nuclear safety authority ASN. International and European studies (JRC, Extern’E) show that this waste management is effective and that health impacts are practically non-existent thanks to the use of matrices, packages and containers, and to storage in pools or dry systems, all of which serve as protective barriers.
Long-lived waste and the protection of distant future generations
Even though radioactive decay steadily reduces the hazards associated with this waste over the very long term, the fact that it is highly concentred (which is also why volumes are low) makes it necessary to take precautions on a timescale of several tens or hundreds of thousands of years, less and less against external radiation, but rather to prevent traces of these products from finding their way into the food chain of people living nearby. Long-term storage can address this concern, but it requires surveillance and maintenance over several centuries: related costs may be low when spread over a century, but cumulative costs over the very long term may become prohibitive. More importantly, we cannot rule out the possibility that, over such long time horizons, societies may be les prosperous than ours and lose the ability to continue to actively manage this waste. Over the past 40 years, these considerations have led to the exploration of two alternative ways to protect distant future generations, through structured research programmes in France (particularly after the Bataille Law of 1991) and the world (IAEA, OECD, European Union):
– Geological disposal: in stable geological environments, it protects the waste packages from external aggression and prevents radionuclides from migrating toward the groundwater and surface. Clay, granite and salt are serious contenders, as they are very abundant in the Earth’s crust at depths of between 300 and 1,000 metres. It was nonetheless necessary to demonstrate and verify the presence of these properties at real sites via drilling and the construction of underground laboratories (in Sweden, Finland, France, Belgium, Switzerland, Germany, Russia, China, Japan…). The research conducted in France at the Andra site in Meuse/Haute-Marne showed that the site was able to fully retain radionuclides for up to a million years, after which only a few traces of radioactivity, less than a thousandth of natural radioactivity, could be detected near any potential outlet. Given the very low volumes in question, the space necessary in terms of depth is not a significant technical challenge, though care must be taken to leave enough distance between packages in the rock so the heat emitted early on by the waste does not reduce the confinement properties of the rock. In sum, from a technical standpoint, the potential for geological disposal in France, or other countries, is not a barrier to a substantial deployment of nuclear power over several centuries;
– Transmutation: the goal is to transform some long-lived waste into short-lived waste or, better yet, into stable (non-radioactive) products, thanks to the development and use of fast neutron reactors (FNR). While research actively conducted in France over the past three decades has delivered some positive results in terms of partitioning the different radionuclides, a solution has yet to be found for transmuting long-lived fission products, knowing that they are the only ones that would still be found in trace amounts around the disposal facility after one million years. The transmutation of minor actinides was successfully done in a laboratory, but a move to the industrial phase would only allow a portion of the actinides to be transformed, and with today’s knowledge, sensitive issues of radiation protection and safety would remain to be addressed. In any event, the mastery of fast neutron technology is a prerequisite, as is the ability to first recycle and then transmute plutonium (a useful step first and foremost to take advantage of its energy potential). Over a reasonable time horizon, we can only hope to transmute a portion of long-lived waste. This makes transmutation at best a complementary solution that would help reduce waste quantities. It would not contribute in a significant way to the health of future generations if geological disposal solutions were adopted with the same confinement properties as have been obtained in recent decades with clay in the Cigeo project; on the other hand, it could be useful if long-term storage is the solution chosen.
The recycling/confinement/storage-disposal solutions used today
Reactors notably require more protective measures in terms of confinement (thickness of concrete,
steel, etc.) and systems for controlling the chain reaction and core cooling; such systems and confinement solutions have been designed and installed over the past several decades in France.
See recent studies by the IRSN on tritium, as well as Louis Patarin (ed.), Le Cycle du combustible nucléaire, EDP Sciences, 2002, pp. 154-155.
See Louis Patarin (ed.), op. cit.
“Radioactive Waste Management”: asn.fr, s.d.
See “National Inventory of Radioactive Materials and Waste”: inventaire.andra.fr.
Stored on-site in a pool for one to three years, then for about 15 years in a pool in The Hague.
See Louis Patarin (ed.), op. cit., p. 153.
Andra, “Le centre de stockage de l’Aube”, May 2014, p. 10
European Commission, “Technical assessment of nuclear energy with respect to the ‘do no significant harm’ criteria of Regulation (EU) 2020/852 (‘Taxonomy Regulation’)”, European Atomic Energy Community,
2021, p. 171
The United Nations Scientific Committee on the Effects of Atomic Radiation (UNSCEAR) estimates that the effective dose for those living near a nuclear power plant (1 km) is about 0.02 mSv/year.
European Commission, ibid., p. 167
French Act 91-1381 of 30 December 1991 on research into radioactive waste management, known as the
“Bataille Law”, after its rapporteur Christian Bataille, and subsequent updates.
For more on these topics, see the examples of StocaMine in France (disposal site in an old potash mine intended for the storage of the most toxic industrial waste), the incidents in old salt and potash mines in Germany (Asse…) and the WIPP repository in the United States (for defence-generated ILW-LL)
Glasses (HLW) would only be placed in geological repositories after being held in storage for about a century, to reduce their thermal load.
French “Act 2006-739 of 28 June 2006 on the sustainable management of radioactive materials and waste”, Official Journal, 29 June 2006.
See Andra, “Dossier 2005 Clay”, June 2005.
a) A number of factors explain why solutions were quickly developed to minimise the effective risks associated with waste when civil nuclear was first being deployed (in 1970-1980)
Starting in the interwar period, when the first applications were being developed for radioactivity in the fields of medicine and industry, a community of scientists, including physicians and biologists, made a decisive contribution to thinking on the topic, and conducted research on the impact of ionising radiation. A commission – which would become the International Commission on Radiological Protection ICRP) in 1950 – was set up as early as 1928, and it began in the 1930s, and especially after the 1950s-1960s, to issue recommendations on protecting workers and communities, proposals that were subsequently adopted by many States. The first phase of research reactors and first-generation power plants in the 1950s and 1960s in the United States, France and the United Kingdom gave structure to the international scientific community working on civil nuclear, which very quickly turned its attention to ways to manage nuclear waste and limit its health impacts in the short and long terms. The key lines of research that would be explored over the next two to three decades were already identified at that time: confinement in long-term storage facilities, in geological repositories under the continents (recommended by the US National Academy of Science as early as 1957), in the stable sedimentary layers (far from faults) of the deep-ocean floor, or dilution in the ocean for the least hazardous waste. The search for ways to reduce quantities at the source also began right away with a focus on the recycling of plutonium and uranium 238 and fast neutrons, knowing that, as early as 1945-1950, it was anticipated that constraints with fissile uranium 235 resources could arise if nuclear was developed at a large scale. The first research fast reactor, Experimental Breeder Reactor I (EBR-1), using a sodium-potassium alloy, was commissioned in the United States in 1951.
In the 1970s and 1980s, the implementation of these waste management solutions was accelerated by the rollout of major second-generation nuclear programmes (most of them using light water thermal neutron reactors) concomitant with the emergence of concerns about the environment. Indeed, this was when the negative side of the industrial boom of the “Glorious Thirty” (1945-1975) began to appear: accumulation, with no precautions taken, of significant quantities of industrial waste, for instance in the Love Canal area in the 1970s, where industrial waste was dumped into an abandoned canal between New York and the Great Lakes with no oversight; dangerous spikes in pollution (Great Smog of London in 1952); and major industrial accidents (Torrey Canyon oil spill in 1967). Some of these events affected public opinion and revealed the dangers posed by the uncontrolled dispersal of hazardous materials.
b) Solutions to reduce the different types of potential dangers posed by waste until the effective risks for humans and ecosystems are negligible
Logically, the search for solutions focused on two goals: reduce potential danger at the source and prevent radionuclides from reaching humans. Reducing the potential danger at the source implies reducing waste quantities. Significant progress has been made on this front, and more is possible in terms of the technological waste from plants or the volumes of certain effluents from the fuel cycle. Recycling spent fuel through a “closed cycle” is also of interest in this regard, as this technique can be used to reduce volumes of HLW, which is the most dangerous, thanks to the use of plutonium and its ultimate destruction.
Existing techniques for stopping radiation from reaching humans either through external irradiation (β- and γ-rays) or internal contamination (notably through food chains, in particular for radionuclides emitting α-rays) involve two steps: immobilisation in suitable matrices-packages and confinement at storage sites that are adapted to the type of waste being stored. These solutions are simple to implement compared with those required in a nuclear power plant in operation1 : rapid decay within the first centuries of the highest emitting β- and γ-rays, absence of pressure, limited thermal level even for HLW (2.5 kW per package then fairly rapid decay in a few decades). Most of what then remains in HLW is α-rays (non-penetrating) associated with actinides that have a low migration capacity. It is even simpler for LILW-SL, which has practically no α radiation nor long-lived fission products, but rather mostly β-γ short-lived products in very low concentrations relative to HLW and that returns to comparable levels of natural radioactivity after 300 years.
The solution involving dilution and dispersion, particularly in the oceans, was abandoned in the early 1980s given uncertainty about the impact for ecosystems and humans of possible reconcentration, for instance due to trophic chains. It is only used today for discharges that pose no danger and are supervised by nuclear safety authorities within the framework of existing regulations.
One example is the regulated quantities of β-emitting krypton 85 with a short half-life of 11 years, a rare gas that is chemically inert (meaning it does not reconcentrate in the food chain, and it stays only for a very short time in the body). Another is tritium, which also has a short radioactive period of 12 years, and is not very toxic since it emits low-energy β-rays and has a very short biological half-life of between 10 and 40 days2.
Specific solutions have been developed for the mining tailings that remain and are left on-site after the extraction of natural uranium. The long-term confinement of tailings is ensured by using a cover that resists erosion to limit the release of radon (a known lung carcinogen) and the infiltration of rain; systems are put into place to monitor potential paths of transfer to humans of uranium and its descendants3.
Nuclear waste management benefits from the ability to accurately measure radioactivity and to trace and monitor (via spectrometry) radionuclides so they can be placed together in packages or separated and distinguished. Waste quantities are very low to begin with and can be further reduced through recycling, including of the radionuclides in spent fuel such as plutonium, provided that technologies like FNR continue to be developed and deployed. The limited number of waste sources and actors involved also makes it easier to follow strict safety procedures, relative to the number of sources and actors involved in industrial and residential waste.
One important characteristic of nuclear waste is that it becomes less hazardous over time. We can identify a turning point at the 300-year mark: at that time horizon, the β-γ rays in short-lived fission products, the most active in terms of radiation, have sharply decreased, and, with them, most of the danger of external irradiation; the low penetrating power α-rays of actinides with low mobility or solubility dominate overall (though a small possibility of external irradiation remains and cannot be ignored, notably because of the braking of β particles in long-lived fission products like technetium 99 that generate X-rays). The main danger then relates to the ingestion or inhalation of these α-emitting products (and, to a much lesser degree, to the ingestion of high-energy β-emitting long-lived fission products, which are less radiotoxic but more soluble and mobile than the actinides). By this time, LILW-SL has reached a level of radioactivity
comparable to natural radioactivity and no longer poses a danger.
On-site storage is an adequate solution for products leaving the reactor and for the initial decades (France being an example), but for the long term, geological disposal should be envisaged as the “permanent passive” solution for waste with a long half-life (and significant activity mainly due to its high concentration). A comparison between long-term on-site storage and geological disposal must take into account several criteria to weigh the advantages of each method of managing waste over very long periods (up to a million years): recurring costs associated with maintenance and renewal of long-term storage facilities over an extended period (dependent on the discount rate applied) relative to an investment cost (geological disposal) borne by current generations; effective protection against potential malevolent acts; and lastly, where long-term storage is concerned, the health risks if a future society is more fragile and no longer has the scientific and technological expertise required to keep facilities from falling into disrepair.
c) France’s example of managing waste for the first 300 years
France has gradually put into place a framework that guarantees a particularly high level of accurate and exhaustive information about and traceability of nuclear waste. This framework was notably organised around:
- The National Plan for the Management of Radioactive Materials and Waste (Plan national de gestion des matières et des déchets radioactifs – PNGMDR), launched by French nuclear safety authority ASN in 2003 and formalised in the Programme Act of 28 June 2006 on the sustainable management of radioactive materials and waste. Published for the first time in May of 2007 and updated every three years under the aegis of the government and ASN, it “establishes a national plan for the management of radioactive materials and waste, inventories foreseeable needs for storage and disposal facilities, and specifies the required capacity of these installations and the duration of storage”4;
- The National Inventory of Radioactive Materials and Waste updated and published periodically by Andra, which provides an annual overview of the quantities of radioactive materials and waste in France, and releases a “forward-looking inventory” every three years with estimated future levels of materials and waste under several different scenarios, depending on the operation and lifetime of France’s nuclear power plants and its long-term energy policy5.
France made the decision several decades ago to gradually move toward a closed nuclear fuel cycle. As of today, spent fuel is reprocessed a few years after it leaves the reactor6, with the fission products and minor actinides to be immobilised in HLW glass packages separated from the uranium and plutonium. The latter is recycled in light water reactors using MOX fuels containing 8% plutonium and 92% depleted uranium obtained from the natural uranium enrichment process at Tricastin. In all, some 120t/year of MOX fuels are used in around twenty 900 MW light water reactors in France. This mono-recycling in PWRs contributes 12 to 13% of France’s annual nuclear electricity production and reduces the country’s uranium needs proportionately.
The MOX fuels obtained from this mono-recycling are placed in storage pools at the facility in The Hague to cool. They remain there for several decades before being reprocessed for recycling in future FNRs, which alone are capable of going all the way to the end of this process of recycling plutonium and all of its isotopes, while allowing depleted uranium (i.e. mainly uranium 238) to be used in iso-generating or breeder reactors over the coming centuries. This assumes that research and innovation will remain robust until the technology is fully developed, and that it will subsequently be rolled out at an industrial scale along with the related tools that will be required: reprocessing of MOX, secure transport of FNR fuels, fabrication of FNR fuels. The partitioning of plutonium-uranium from fission products and actinides and then plutonium alone in The Hague (Purex procedure), the fabrication of the MOX fuel at the Melox factory near Marcoule, and the development of the glass matrix for the HLW, constitute a unique set of technological innovations, industrial expertise and human skills for the future. While some ILW-LL is found both with closed and open fuel cycles (waste associated with activation products in vessel internals, for instance), a significant share relates to the reprocessing of fuels in The Hague (effluents from the partitioning process, hulls and end-caps from fuel assemblies cut during reprocessing…).
Solutions for confining and recycling HLW are conceptually simple but nonetheless require significant technological and organisational innovation. While this is clearly the case for HLW, to a lesser degree, the same can also be said of ILW-LL and LILW-SL: development of bitumen or cement matrices, compaction of hulls and end-caps to reduce their volumes, significant decrease in radioactivity in liquid or gaseous form by EDF (reduced by a factor of 10 between 1990 and 1998) and at The Hague facility (reduced by a factor of more than 50 between 1984 and 1998)7 , operations at the incineration and fusion plant of metallic waste (Centraco) to reduce volumes prior to cementation of LILW-SL, etc.
Once these different categories of waste have been separated, quantities have been reduced, and the waste has been placed into appropriate matrices, packages and containers, the last step in the waste management and confinement process is to choose the appropriate storage or disposal option:
- VLLW: the surface storage site reserved for this waste, the Centre industriel de regroupement, d’entreposage et de stockage (Cires), is located in Morvilliers, in the Aube department; this waste has a radioactivity level close to natural radioactivity, and its volumes come mostly from nuclear power plant dismantlement;
- LILW-SL is stored at the Centre de stockage de l’Aube (CSA) in Soulaines-Dhuys, which opened in 1992, taking the baton from Centre de stockage de la Manche. This “basic nuclear installation” (BNI) is operated by Andra under the supervision of ASN. It is currently authorised to receive 1 million cubic metres of waste packages and has a footprint of 95 ha, of which 30 ha are reserved for the storage of LILW-SL. At the end of 2016, the centre was operating at about 36% of its total authorised capacity. This being a surface storage facility, the annual radiation dose must be kept as low as possible and cannot exceed 1 mSv for the population. After it ceases to operate, the CSA will continue to be monitored for at least 300 years, until it can remain safe without any human intervention because the waste will have the same level of radioactivity as natural radioactivity. Tests performed have proved that the site is indeed safe today: according to Andra, “the radiological impact of the CSA for the year 2012 is estimated at 0.00097 microSieverts (µSv), which is more than 100,000 times lower than the regulatory limit and than the average impact of natural radioactivity in France8”;
- LLW-LL is currently stored while awaiting subsurface or deep storage solutions. It is present in limited quantities and mostly results from the legacy generation I nuclear reactors (natural uranium-graphite-gas reactors, or UNGG);
- ILW-LL and HLW are stored while awaiting a very long-term disposal solution. Most HLW, which is the most radioactive, is stored at The Hague, in wells ventilated through natural or forced convection to evacuate its residual heat, under a layer of concrete sufficiently thick to ensure protection against penetrating γ-rays, especially during the first decades. Here again, exposure of workers and nearby communities is closely monitored by the ASN and remains negligible. This storage solution is intended to last for a few decades, and may be renewed if necessary, depending on the strategy adopted for the long-term management of this waste
Such efforts to research and implement innovations to limit the health impact of ionising radiation from the civil nuclear industry, particularly from waste management, have been quite satisfactory in France, but also internationally. A major study conducted in 2020 under the aegis of the European Union showed that the average impact of the nuclear power industry in France on the entire population was 0.0002 mSv/year9, which is some 10,000 times below natural radioactivity10. This study summarised previous research on the topic and concluded by showing “an absence of significant impact on health or the environment”, confirming compliance with the fundamental criterion “Do No Significant Harm” (DNSH): “In the light of the above analysis it can be concluded that activities related to the storage & the disposal of technological & radioactive waste, as well as spent nuclear fuel do not pose significant harm to human health or to the environment11”.
d) Very long-term solutions (beyond 300 years) for HLW and ILW-LL and the benefits of geological disposal: France’s approach
In the 1980s, a focus at the international level on three areas of research:
long-term surface storage, geological disposal, and transmutation
We thus already have access to solutions that are safe for all types of waste, whether disposal or surface or subsurface storage, on a time horizon of a few decades or centuries. The solutions available on these timescales are sufficient for the most part (in volume terms), but not for spent fuel (open cycle) or HLW (closed cycle), with the related ILW-LL, as the latter are characterised by a high level of activity beyond two or three centuries and for up to a few hundred thousand or million years. This raises questions about whether societies in the distant future will be able to maintain and renew long-term surface storage facilities. It might also be “collectively” more economical to invest once and for all in a sustainable, passive safety solution such as geological disposal. Beyond the exploration of the relative advantages of long-term storage and geological disposal, research is being done into technological innovations that would make it possible in the not-too-distant future to transform some of this long-lived waste into short-lived waste or, better yet, into stable elements, reusing it where possible to produce energy (partitioning and transmutation).
All three approaches – long-term surface (or subsurface) storage, geological disposal and partitioning and transmutation – were identified in the 1980s in different countries with nuclear programmes. In France, the Bataille Law of 199112 gave structure to the research that was to be conducted in these three areas over the following 15 years, placing it under the supervision of policy- and lawmakers (with scientific support from the National Assessment Commission, or CNE).
The initiatives taken as part of the Bataille Law of 1991
The first priority was to take into account the very political dimension of the debate on nuclear waste management at two levels:
- local: local residents have legitimate questions about the short- and medium-term impacts of living near a geological disposal site (safety over decades of operation, effects on the area’s image…);
- national: waste management decisions make us accountable to distant future generations, and thus depend in part on our views of how society might evolve, what progress we believe could be made and the role of science, and the future and role of nuclear power in the country’s energy mix.
Another goal was to take time to reduce scientific and technological uncertainties and go from a concept on paper to experiments in the field and “at scale” before planning industrial implementation. What can we realistically expect the future to hold for transmutation, at what time horizons, and what long-lived products might be transformed at an industrial scale? As regards geological disposal, the first step was to hear the concerns of potential local communities, reflecting in part past incidents and accidents at certain industrial or nuclear waste storage sites, especially former mines (salt, potash…), but also to acknowledge citizens’ questions about whether scientific claims regarding the efficacy of the geological barrier over time horizons as distant as one million years can be believed.
Analysis of past incidents shows that they occurred during the operational phase, when the repository was not yet closed, and were caused by packages containing products the proximity of which can cause fires or explosions (lack of rigour regarding traceability and verification, packages and matrices that are ill-adapted to the products they contain…). This risk is even greater if operations entail the presence of fire or explosion initiators (electrical sources, ventilation systems) that can come into contact with the waste before the disposal cells are sealed to isolate it. Moreover, there are numerous openings when a repository is built in an old mine, representing weaknesses in confinement vis-à-vis the circulation of water that can attack the packages and disperse certain toxic products (based on the water cycle). If, moreover, recoverability has not been planned for this phase of operations, then the management of incidents that are not very dangerous initially can become sensitive.
The simple but important conclusions we can draw from all this are that we must13:
- Ensure the existence of a solid regulatory framework as well as competent supervisory authorities and operators;
- Guarantee the verification and traceability of products and packages (in particular, refer to the Andra inventories and the PNGMDR mentioned above);
- Verify the robustness of matrices and casks before the cells and tunnels are closed off and sealed to be watertight;
- Disrupt the host rock as little as possible, by avoiding using former mines, limiting unnecessary intrusions and drilling in the rock, and closing off openings (cells, tunnels) as quickly as possible;
- Allow for package recoverability as long as the cells are open, here again making sure they are closed as quickly as possible to protect the packages from external aggression.
The last key priority pursued over this period (starting in 1991) was to develop more robust arguments over unprecedented timescales, spanning hundreds of thousands of years, or even a million years, concerning geological confinement. This required:
- Presenting the different timescales explicitly and clearly: the timescale of civilisations (from centuries to millennia), the timescale of the evolution of the thin biochemical layer on the surface of the Earth and that of its climate with cycles of glaciation and deglaciation (50,000- or 100,000-year timescales) and soil erosion associated with the water cycle (affecting the subsoil to 100 or 200m), the timescale of the evolution of the human species over a few hundred thousand years (Homo Sapiens appeared 200,000 to 300,000 years ago), the radioactive decay periods of most long-lived radionuclides the activity levels of which fall to low levels within a few hundred thousand or a few million years, and the specific timescale of the history of geological layers situated far from the fracture zones associated with tectonic plates and which have been stable over several hundred million years (such as the Callovo-Oxfordian claystone);
- Backing up these studies with in-situ or in-laboratory experiments to test the specific properties of the rock at a given site, for instance to confirm low water circulation, this being the biggest threat, and to test the migration speeds of the different products, all with support from the different relevant fields of expertise (geotechnical, hydraulic, chemical, biological, numerical simulations, etc.) through organised multidisciplinary scientific and technical research.
To successfully pursue research into all three types of solutions, a specific governance structure was put into place in 1991 under the aegis of Parliament, with participation by the OPECST. The concerns expressed by the different regions were of crucial importance (see, for instance, the role played by local information and monitoring commissions and committees). The ASN, with technical support from the IRSN, played a central role when it came to compliance with safety and radiation protection standards. All public agencies involved – CNRS, BRGM, CEA, IRSN, Andra, Orano and EDF– contributed to this multidisciplinary scientific and technical research, with scientific oversight, also multidisciplinary and independent, by the CNE.
The debate about reversibility
Reversibility became a central topic in the debate about nuclear waste management starting in the 1980s, and it is useful to clearly explain the different meanings that can be given to this term:
- First, it translates the idea that it is not necessarily urgent to make decisions about how long-lived nuclear waste will be managed in future. Indeed, this waste is currently being safely managed in temporary storage, with no time constraints over the short or medium terms. This creates space to keep our options open while we reduce uncertainty through research, before taking educated and informed decisions (vision consistent with the precautionary principle as introduced in the Charter for the Environment in France), and also leaves time for conducting vital consultations with stakeholders (it was the initial assessment in 1990-1991 that shaped the research work done over the following 15 years);
- Second, it can refer in a more concrete sense to the actual recoverability of the packages, notably in the event of a problem, to ensure efficient management of incidents while the repository is in operation and the cells are open;
- Third, it conveys the idea that the process underway does indeed involve phases with the government intending to maintain control over major decisions (choice of site, decision to begin construction, date of nuclear waste storage, date when the repository will be closed…). These decisions can thus be shaped by possible changes in the country’s nuclear strategy and, consequently, the inventory of waste to be stored (nuclear phased out or not, closed cycle with FNRs or open cycle). This dimension was agreed upon in 1991 and remains relevant today;
- The last meaning assigned to the notion of reversibility is closely related to the possible conflict between two end-goals (or values): “passive protection” of distant future generations versus leaving those generations the “freedom of choice” to select a waste management solution. Long-term surface storage (together with the transmission of a body of knowledge relating to the different solutions) represents the “freedom of choice” solution, and closed geological disposal (after waste quantities have been reduced) represents the “passive protection” option.
With the first two meanings, which focus on present generations, reversibility is bound to gradually become a nonissue as decisions are taken that rule out some options, for instance if construction starts on a disposal site or its closure is initiated. As regards the last meaning assigned to reversibility – letting future generations choose their own solution, whether sustainable or not – it is important to bear in mind that successive opinions from the ASN (since 2006) have underscored the need to seal the cells (once filled)14 and shut the repository (also once filled) for confinement to be effective. In rather blunt terms, an open geological repository is nothing more than long-term storage located a few hundred metres below ground. And, except in a few specific situations, the protection afforded distant future generations is no better than with long-term surface storage. One can even ask if it does not create new fragilities due to the complexity of the design. It should also be recalled, however, that irreversibility and reversibility are relative terms when it comes to geological disposal: even an entirely closed repository remains accessible through drilling and mining techniques. Gaining access for recovery becomes more difficult over time as the cells are sealed, the repository is closed, and the plasticity of the host rock causes it to close in around the cells and packages, but it will never be totally impossible for humans if they have scientific and technological tools that are better than or at least as good as ours today.
Conversely, protection against natural elements and the effects of time is ensured in a passive manner once the cells and repository are closed. The choice between long-term storage and geological disposal, based on the weighting assigned to two objectives – protection or freedom of choice – must thus be made based on reflection and discussions that take into account these observations as well as a prospective analysis of future societies and the resources of ethical philosophies, these being important points that will be discussed in Part IV.
The “benchmark solution” per the Law of 200615: moving toward geological disposal
The Law of 2006 (and the ASN opinion of February 2006 accompanying it) drew lessons from the 15 preceding years of research (a major undertaking supported by just over 3 billion euros of investment):
- The recycling of plutonium and depleted uranium was to be ensured via the industrial development of fast neutron technologies over the following decades. Partitioning and transmutation beyond plutonium would not be ready for an industrial rollout over this timeframe, and would in any event not have allowed the recycling of existing vitrified HLW or the transmutation, in the near term, of ILW-LL and the totality of HLW. In other words, this remained an interesting avenue of research for reducing quantities, but it did not eliminate the need for long-term storage or sustainable geological disposal solutions;
- Andra’s “Dossier 2005 Clay” report highlighted the qualities of the future Cigeo facility, where geological conditions were very favourable16. It is now considered the benchmark solution insofar as long-term storage does not appear to be a robust solution for protecting future generations if, in the long run, societies become weaker institutionally, technologically and economically (ethical reasoning regarding our responsibilities). The new objectives for the next decade were to build on existing research while preparing to launch an industrial project so the site could accommodate HLW and ILW-LL. These targets were reiterated in the law of 2016 with a cost estimated at 25 billion euros. Most of the steps required to move this investment forward have been taken as of today: the application for a declaration of public utility is being reviewed (it was submitted in August 2020), paving the way for public authorities to commit to the investment.
In sum, several decades after initiating this process, France is clearly moving toward opting for geological disposal of its long-lived waste. It thus appears that 15 years of research (1991-2006) into these three options have led to the adoption of a solution that combines all three: transmutation to reduce the quantity of waste to be stored, storage to manage the thermal output of waste and await the completion of consultations and research, and geological disposal.
These approaches have been the subject of discussions and research in most countries with civil nuclear programmes. International institutions like the IAEA, the NEA-OECD and the European Union have also contributed to convergence on this shared vision of the best tools for managing long-lived waste, with geological disposal determined to be the benchmark solution.
Geological disposal in France and the world
For this portion, see Andra, “Andra research on the geological disposal of high-level long-lived radioactive waste. Results and perspectives”, June 2005, and its updates, particularly “Study of direct disposal of spent fuel from pressurised water reactors and fast neutron reactors”, 3 July 2013. Also see CEA, “Advances in research on partitioning-transmutation and plutonium multi-recycling in fast neutron reactors”, June 2015.
See Andra, “Dossier 2005 Clay”, www.andra.fr. Also see Ghislain de Marsily, “Enfouissement des déchets nucléaires en formation géologique”, in R. Turlay (ed.), Les Déchets nucléaires. Un dossier scientifique, Les Éditions de physique, 1997, chap. 10, Castaing report (1983), as well as the Goguel report, “Stockage des déchets radioactifs en formations géologiques”, CIDN, 1988
See “FSR-III.2.f (repealed by the Safety Guide of 12.02.08 for the deep geological disposal of radioactive waste) of 01/06/1991”, asn.fr, 6 October 2009
See Andra, “Andra research on the geological disposal of high-level long-lived radioactive waste. Results and perspectives”, June 2005, p. 12. For more in-depth information, see Andra, “Dossier 2005 Clay”, tome “Phenomenological Evolution of a Geological Repository”, June 2005.
See Andra, “Dossier 2005 Clay”, www.andra.fr. Also see annual reports from French National Review
Board CNE, Andra’s “Safety Options Reports”, and IRSN analyses of the quality of these safety studies, which were also validated by international scientific assessments.
The different calculations may therefore change depending on the assumptions and margins applied in
the studies. The order of magnitude of 15 km² for the footprint of the reference inventory alluded to later in the text appears to be a robust size.
See Andra, “Dossier 2005 Clay” and its updates, particularly Andra’s technical document entitled “Étude relative au stockage direct des combustibles des réacteurs à eau pressurisée et des réacteurs à neutrons rapides”, 3 July 2013. Also see CEA, “Advances in research on partitioning-transmutation and plutonium multi-recycling in fast neutron reactors”, June 2015
See, in particular, the safety options report submitted for the project in 2017 and its review that same year by IRSN (the technical support of the ASN) and the application for a declaration of public utility submitted by Andra in 2020.
See the Extern’E study and the JRC study for the European Union.
a) The different geologies available internationally, sites in France17
The expectation with final disposal of long-lived waste in deep geological repositories is that it will provide protection and retention for a very long period (100,000 to 1 million years, the time required for radioactive decay) by protecting the waste packages from external aggression and preventing their contents from escaping. This waste is only stored once every effort has been made to reduce its volume and concentrate it (this concentration is in turn what makes it potentially dangerous even over the long term). One advantage of this passive protection is that no human action is required once the disposal site is closed (contrary to long-term storage). External aggressions can come from humans (malevolence) or from nature: climate change and glaciation and deglaciation (which explains why a depth of more than 150-200m is required to avoid being subjected to them), instability of geological layers, circulation of water potentially carrying products that could corrode the containers… If, after a process spanning a very long period, the “engineered barrier”, the package and the matrix are deteriorated, then the characteristics of the geological layers must, for the time required, prevent the migration of the radionuclides toward the water tables and the surface (these are the successive “barriers” underpinning the “defence in depth” approach to safety).
Geological layers that remain stable over the very long term and are susceptible of displaying these properties are fairly prevalent and diverse. Granite, salt and clay are the top candidates for disposal sites:
- Granite has very good geotechnical characteristics. Given its high mechanical resistance, large cavities can be built with no need for retaining structures, contrary to some other rocks, which are more plastic and subject to creep like for instance claystone and especially rock salt. Constructing this type of disposal site is easier, as is recovering packages. Thanks to its good thermal conductivity, the waste with the highest thermal output can be transferred there sooner. On the other hand, fissures (fractures) may appear in the granite, meaning that, while it is not very permeable, some water circulation cannot be ruled out. Beyond seeking out granite with few “fissures”, this drawback may be compensated for through initial barriers (packages and engineered barriers) that are more impermeable to water, and more confining for radionuclides: containers of pure copper (highly resistant in a reducing environment of granite) and coating in bentonite (clay) as an engineered barrier around the packages in the cells (such protections are even more useful if used with spent fuel rather than glass matrices, which are more resistant to water aggression). Such solutions are being envisaged in Finland and Sweden, countries that are planning to dispose of spent fuel (open cycle) in the granite of the “Scandinavian Shield”;
- Salt, conversely, rules out a priori the presence of water (prior to excavation by humans) and, since it is very plastic, it compacts rapidly around the packages and hermetically seals the cells and tunnels. This quickly makes the packages hard to access for recovery. Salt is a good thermal conductor. On the other hand, it could be a potential target for future explorations since it is one of the resources, or located close to the resources, sought after by humans. It can also be weakened by too much drilling and the resulting potential infiltration of water (this is the problem encountered in some old mines used for disposal);
- Clay is not as good a thermal conductor so it is preferable to allow the waste with the highest thermal output (HL) to cool for a few decades (at least 60 years) in storage. The goal is to limit the temperature inside the rock to prevent degradation of its confinement qualities. It has less plasticity than salt but requires more retaining structures than granite. Recovery of packages is possible over a reasonable time horizon. This is an environment saturated with water in micropores but no circulation between pores; it is thus very impermeable and, in most cases, has very good radionuclide retention characteristics, especially α actinides. And, a priori, clay is not a resource that justifies drilling.
Whatever rock is used, it is indispensable to verify, on-site if possible, the absence (or at least the limited presence) of fractures and significant hydraulic gradient, as well as the low permeability and robustness of the rock, during geotechnical work. Specific studies are thus all-important. Very significant progress has been made in recent years in understanding diverse geologies, particularly as regards their stability, depending on how far they are situated from zones where tectonic plates meet and on their various properties (thermal conductivity, reducing or oxidising environment, hydraulic gradients, impermeability…). The observation of natural analogues has supported these research results, particularly that of uranium mines and the discovery of Oklo, a two-billion-year-old working “natural nuclear reactor” in Gabon.
Based on the characteristics of the site, the three confinement barriers – geological, “engineered” and the package-matrix – must be thought out as a whole and in a coherent manner. Here again, considerable research has been conducted at sites in different parts of the world over the past five decades, with international coordination of the scientific community and the various relevant disciplines (around the European Union, the OECD-NEA, and the IAEA), to compare viewpoints and test analyses.
Different studies conducted in France over the past four or five decades have helped identify a large number of granite, salt or clay sites that would in theory be favourable from a technical standpoint. In 2005, the last Andra study on granite showed advantages for 78 areas in France with a footprint of more than 20 km² each, in the Massif central and Armorican Massif, away from major faults. These granitic sites have significant thickness of between 300 and 1,000m. Here is an example of good technical potential the cumulative capacity of which would be at least one order of magnitude greater than the “Transposition Zone” (250 km²) of the Meuse/Haute-Marne site selected for the Cigeo disposal facility in France. It is also important to remember some of the areas that were explored in the 1980s: clay in the Laon region (Aisne), salt in the Bourg-en-Bresse region (Ain), granite in the Niort region (Deux-Sèvres), shale (ancient clay, folded and very thick) in the Angers region (Maine-et-Loire) and, more recently (late 1990s), clay in the Gard, near Marcoule, and granite in the Vienne. The studies and research conducted in these areas were not completed, in most cases more for reasons related to political will or acceptance by local populations than to scientific or technological factors, so it is interesting to keep in mind the technical potential they hold in each case, subject to rigorous analyses being conducted at the sites18.
We discuss below how other countries, for the same reasons, also hold significant potential in favourable geological layers in clay, granite or salt.
b) Geological disposal in France: the Cigeo clay repository project
Let us take a closer look, for illustrative purposes, at the rock and the site selected by France, the Callovo-Oxfordian claystone at the confluence of the Meuse and Haute Marne, and at the science- and policy-based safety measures taken to test and qualify the future site of the Cigeo project. A negligible very long-term impact on health and the environment Fundamental Safety Rule III.2 (FSR III.2) on the final disposal of radioactive waste in deep geological formations was issued in 1991 by the ASN19, which, as discussed above, has since been very involved in geological disposal safety issues and the choice of a permanent solution for long-lived waste. This FSR sets forth the key criteria sought in a deep disposal site:
- absence of seismic risk over time;
- absence of significant circulation of water within the disposal facility;
- rock that can be drilled to install the facility (wells, tunnels, cells);
- confinement property vis-à-vis radioactive substances (related to the choice of the matrix, the package-container, the sealing and coating materials);
- sufficient depth to keep waste away from various forms of aggression;
- absence of mineable rare resources nearby.
To uphold this Rule, the ASN set an extremely cautious and stringent objective in terms of effective dose over the very long term for the individuals most impacted near potential outlets, below 0.25 mSv/year, which is a tenth of natural radioactivity and a quarter of the standard currently applied to protect present generations from ionising radiation in general (1 mSv).
The Meuse/Haute-Marne site meets all these criteria and appears, after three decades of research, particularly via the underground laboratory set up in 2000, to be one of the most favourable geologies in France: this mudstone, more than 130m thick and located at a depth of between 400 and 600m, is 155 million years old. The disposal cells that will host the most radioactive waste – HLW packages – will be micro-tunnels 100-150m long with a diameter of 60-70cm, each containing a few packages. These packages, standard vitrified waste canisters (CSD-V), contain HLW incorporated into a glass matrix with great confinement power lasting several hundreds of thousands of years under geological storage conditions. These “glasses” are poured into stainless steel canisters, which are in turn inserted into cylindrical overpacks that are made of low carbon content steel and 6.5 cm thick to resist corrosion and ensure watertightness at least for the first 4,000 years.
If, after these periods of time, a radionuclide began to migrate outside the package into the clay, it could “enlist the services of” a droplet of water for transport. Yet in this type of clay, a droplet of water only advances by a few centimetres in 100,000 years20. Moreover, the chemical compositions of the rock and its interstitial water will lead to the capture (precipitation, adsorption…) of most of the radionuclides. As a result, actinides, being heavy atoms, barely migrate at all over a million years (due to their chemical properties, they precipitate in a reducing environment), and only a few of the most soluble fission or activation products (iodine 129, selenium 79, chlorine 36) could be found in trace amounts after one million years in the layers around this Callovo-Oxfordian claystone, though they would have no impact on humans or the environment.
Researchers arrived at these results by constructing normal or altered evolution scenarios (human intrusion, for instance) and calculated the individual doses of the group constituted by the individuals most exposed, those living near the possible potential outlets of these geological layers, adopting the less favourable (“penalising”) modelling whenever uncertainty exists. Even with these pessimistic assumptions, the traces that appear only after one million years for these individual doses are below 0.0025 mSv for HLW and ILW-LL packages, which is 100 times lower than the standard set by the ASN, and below 0.025 mSv where spent fuel is involved (10 times lower)21.
There is sufficient technical storage potential in the “Transposition Zone” for a few centuries of nuclear power generation.
These results, obtained through experiments in the underground laboratory and related modelling, can be transposed to an underground area of 250 km², the “Transposition Zone”. The Cigeo disposal project planned for this site is currently designed to only house the HLW and ILW-LL already produced by existing and past facilities – including research tools of the 1950s-1970s and the first-generation UNGG nuclear power plants that have been decommissioned and are being dismantled –, as well as the waste that will be produced by nuclear power plants that have been authorised and are under construction (EPR in Flamanville, ITER, Jules Horowitz research reactor), based on the assumption that the reactors will remain in operation for 50 years on average. This represents about 10,000 m³ of HLW and 75,000 m³ of ILW-LL, referred to as the “reference inventory” for the Cigeo project. Though HLW volumes are ten times lower than for ILW-LL, the former requires the most space underground in cells because of its “thermal output”: there needs to be sufficient space between packages to avoid overheating the clay (below 90 °C) during the first centuries and to preserve the rock’s confinement properties.
In 2009, Andra proposed for the Cigeo project an underground area of 30 km², the “zone of interest for detailed reconnaissance” (ZIRA), located within the “Transposition Zone”, based on a consultation with local residents and elected officials, to ensure that the “reference inventory” could be stored there and that there would in theory also be room for what is called the “reserve inventory”, i.e. the spent fuel that would remain (and be requalified as waste) if a move was made to switch to an open fuel cycle and keep existing plants in operation with no reprocessing, or if a decision was made to shut down nuclear power plants after 50 years of operation, as well as the additional HLW and ILW-LL that would be generated if the life of existing reactors was extended.
To estimate the storage potential of the Meuse/Haute-Marne geological site, beyond the “reference inventory”, which by definition does not take into account potential future generations of nuclear power plants, it is necessary to discuss a few orders of magnitude depending on the assumptions made about the future of the French nuclear fleet: open or closed fuel cycle, nuclear phased out or not. These orders of magnitude are reconstructed from data found in a variety of documents22. This important topic would deserve to be presented in an accurate, rigorous and concise way, which unfortunately is not the case today.
Under all scenarios, over one generation, 50 years, of a nuclear fleet producing 400 TWh/year, some 2 km² would have to be mobilised to dispose of the ILW-LL produced, and it would take another 1 to 3 km² for a “common base” (other waste, shared access tunnels…). The area in depth required to dispose of HLW (all-important, as we have seen, due to its thermal output) would be close to 4 km² for 50 years of operation with France’s existing capacity. If the same fleet (producing 400 TWh/year) was operated with an open fuel cycle, the depth required would be closer to 10 km² for the disposal of all spent fuel produced over 50 years. If reprocessing continued until existing plants reach the end of their service life, and fast reactor technology was not developed to continue to recycle the spent MOX fuels resulting from the mono-recycling of UOX in pressurised water reactors, then sufficient storage would be needed not just for HLW but also for the spent MOX fuel
produced over the life of the fleet. The area in depth required would in this case be only slightly lower than with an open cycle, at around 8 km².
We can thus see that, from a strictly scientific standpoint and not considering acceptability to local communities, there is sufficient depth at the Meuse/Haute-Marne geological site for several generations of nuclear power plants, even with an open fuel cycle, if we count not just the 30 km² of the ZIRA, but also the 250 km² of the Transposition Zone. For a single generation (50 years of production) of existing nuclear power plants (400 TWh/year), the depth required, for all of the waste in question, would be close to 15 km² for a PWR scenario with an open fuel cycle or a PWR with mono-recycling, or 7 km² (equivalent to a 2 km x 3.5 km rectangle) for a scenario with a FNR and closed cycle, representing a footprint of 150 km² for 500 years with an open cycle or 70 km² with a closed cycle.
Mono-recycling alone has limited benefits in terms of reducing waste volumes and the space taken up at the disposal site. On the other hand, the “transmutation-recycling” of the main actinide, plutonium, via fast neutron reactors, would help reduce the underground areas required by at least a factor of 2. It should also be recalled that if HLW was stored for 100 or 120 years instead of 60 years to allow it to cool prior to disposal, the depth required would be nearly halved, implying a gain of a factor of four relative to an open fuel cycle (or mono-recycling alone); therefore from a technical standpoint, the storage potential of this site would reach more than a millennium. The next step after transmutation is the fission of the americium. This would reduce the surface area required by a further third, provided that it is done over the long term, which is not without benefits, but clearly not as transformative as the two previous steps (which are of course also easier to execute)23 .
Cigeo: an industrial project awaiting a policy decision. Its costs are limited considering nuclear power generation costs. Appropriate governance is required for its successful implementation.
The Cigeo project has already been finalised from an industrial standpoint and in terms of the main safety options24. What remains now are to secure regulatory authorisations and the authorisation decree from the government, after which work can start, once the decision to commit is taken by public authorities based on their strategy. The national and local consultation process has been particularly intense in recent years. Andra has acquired industrial design and execution skills, with notable help from producers of waste (EDF, CEA, Orano), adding to its expertise in research and safety. As a result, all that remains, essentially, is a policy decision. The footprint below ground would be around 15 km² (equivalent to the footprint of the Paris-Orly airport), enough to accommodate, if necessary, not only the HLW and ILW-LL from existing light water reactors, but also “legacy” waste (research, military, UNGG reactors, etc.). The project objectives are clearly centred on this “reference inventory” (expanded, if necessary, only to include the “reserve inventory”, notably if nuclear generation and reprocessing were gradually phased out and the spent fuel needed to be stored). This is why, for several years, there has no longer been any talk of the below-ground footprint necessary to continue the nuclear programme sustainably, as mentioned above.
If the project is approved and implemented, it will have a lifespan of about 100 years: close to 15 years for the pilot phase and construction of the main infrastructure, from 2025 to 2040; then, between 2040 and 2100, disposal of ILW-LL; and from 2080 to 2140, disposal of HLW (the tunnels and cells would be drilled progressively, based on capacity needs). HLW is disposed of later because it must first cool for at least 60 years in storage. The cost of the project is close to 25 billion euros, of which around 10 billion would be spent during the initial construction phase in 2025-2040; capital expenditure would account for half of the total 25-billion-euro cost (the rest would go for operations-maintenance, taxes, and costs associated with the contracting owner and project managers). Gross costs, accumulated over more than 100 years without being discounted, and divided roughly by 400 TWh a year over 50 years, work out to around €1/MWh, while baseload electricity prices (such as the full cost per megawatt hour of nuclear) are close to €50/ MWh. In other words, the difference is no more than a few percentage points (coherent economic calculations taking into account the temporality of choices yield even lower results with a wide range of discount rates). In sum, disposal costs do not threaten the competitiveness of nuclear, contrary to the CO2 price with emitting generation units, which increases the full cost of electricity from coal by a factor of 2 or more and adds at least 50% to the cost of electricity from gas (with a CO2 price of around €50 to €100/t). Moreover, with a broader vision of the externalities of energy-related businesses, taking into account the economic cost of the health impacts of different types of pollution from fossil-fuel power plants (particulates, SO2, NOx, toxic chemical waste…), it is at least one order of magnitude higher25. The cost of disposal is thus low in relation to nuclear power generation costs (and the cost of externalities for other energy technologies), though it
is high in absolute terms, particularly when it comes to the cost of building common infrastructure during the pilot phase (surface infrastructure, waste transfer ramp to tunnels and cells, etc.).
The bottom line is that this is a major investment project, one that is complex notably due to its duration (around 100 years), the multiple decision points, and, mostly importantly, because of its governance and the number of public and private players and associations involved, as well as the need for continuity of expertise over time. Because of this complexity, the project must have clear governance, effective industrial oversight, and a decision and accountability chain that is visible and simple, if we are to avoid cost overruns and delays, especially if our priority is delivering a safe structure for future generations.
The Cigeo disposal centre
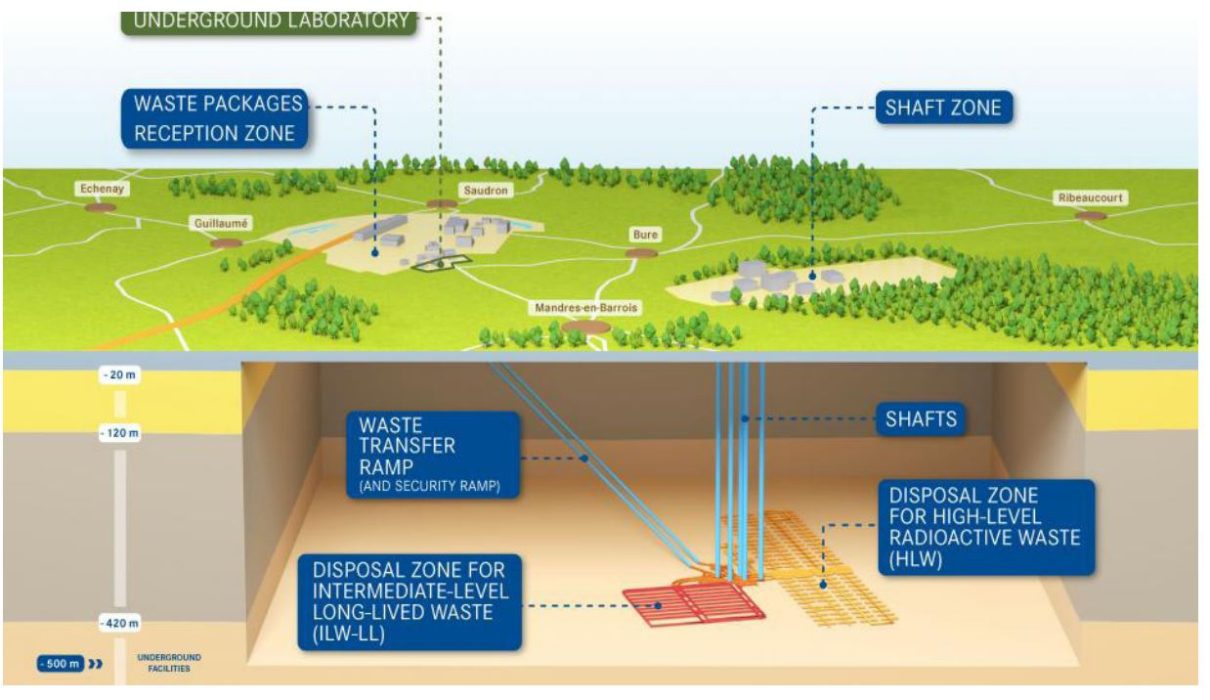
Source :
https://international.andra.fr/projects/cigeo/cigeos-facilities-and-operation/project-siting-and-facilities-overview
IAEA, “Nuclear Technology Review 2020”, p. 23.
Nathalie Steiwer, “Nucléaire : l’Allemagne n’aura pas de site de stockage avant 2030”, lesechos.fr,
19 August 2021.
Ibid.
Except in some small countries that do not have favourable geologies and cannot enjoy the economies
of scale that come from building sites that can accommodate sufficient volumes of waste. Once several geological repositories will have been built in the world, the question of international solidarity will arise as effective solutions are sought for all countries wishing to use civil nuclear
c) Overview of geological disposal across the world
All countries with large civil nuclear programmes take the same general approach that includes long-term geological disposal to protect future generations as well as interim storage solutions for more or less long periods. This convergence was achieved thanks to the organisation of joint research programmes between the different countries around the European Union, the OECD (NEA) and the IAEA.
This shared vision is nonetheless reflected differently in different countries, depending on individual energy and nuclear strategies (open or closed cycle, plans to eventually phase out nuclear…), the geologies selected, and the ability to organise quality consultations and debates at the local and national levels. It is possible to create a typology by taking a few countries that are representative of this diversity and classifying them according to their nuclear strategy: open cycle, closed cycle, plans to phase out nuclear or not.
Open fuel cycle
- Finland and Sweden have made the most progress in their process: certification of a site in each country for spent fuel; commitment from policymakers and construction underway in Finland, awaiting a government decision in Sweden. Both countries have opted for disposal in granite (characteristic of the Scandinavian subsoil), which would allow for provisional recoverability of packages (but, a priori, only before the bentonite is put into place), an interesting feature since it is very possible that a solution will be found to tap the energy potential of the spent fuel (plutonium) over the coming decades to allow mass production of CO2-free electricity (subject to the maturity of FNR). To protect spent fuel packages from water circulating in the faults in the granite (in theory few in number since the sites are relatively free from fractures), Finland and Sweden are using copper canisters and engineered barriers in bentonite (clay).
- The United States has opted for an open fuel cycle (decision made by Jimmy Carter in 1979, as part of the non-proliferation policy). The country already has a geological disposal repository in WIPP (Waste Isolation Pilot Plant), which was brought into service in 1999 in a salt dome to house defence-generated ILW-LL. Since the early 1980s, the country has been envisaging disposing of spent fuel from civil nuclear power plants in Yucca Mountain, in the Nevada desert, in the volcanic “tuff” on the side of the mountain. Progress is for now being held back for political reasons, as the site, though protected by the desert, does not present the best impermeability properties relative to other possible parts of the American subsoil. In the meantime, spent fuel is being kept in long-term dry storage at reactor sites under the close supervision of the Nuclear Regulatory Commission (NRC).
Closed fuel cycle
- France is today the most advanced country regarding reprocessing waste at an industrial scale and with the Cigeo project.
- Russia is the most advanced country with FNR (after France recently scrapped its Astrid project, having in recent years closed its Superphénix 1 200 MW and Phénix 250 MW nuclear plants). The country is developing reprocessing and vitrification technologies but has not yet matched France’s industrial expertise in these areas, though it is working actively to do so. Some 30 potential geological disposal sites have been identified in several regions (granite in the Kola Peninsula, Siberia, the Ural, the Volga region…), and the agency in charge of waste management is envisaging creating an underground laboratory near Krasnoyarsk (4,000 km east of Moscow, in central Siberia) before building a geological repository toward 2035-2040.
- China got a later start but is quickly catching up in all aspects of the closed fuel cycle, with projects for fast neutron reactor prototypes of nearly all kinds. The country is also making progress on geological disposal of HLW, as the IAEA reports: “In China, final authorisation was given on 6 May 2019 for the construction of an underground research laboratory in the Beishan area of the Gobi Desert (granitic formation). It is intended to investigate the local granitic rock’s suitability for hosting a deep geological repository for HLW. It is planned to be constructed at a depth of 560 metres and will be open to requests from other Member States interested in conducting and participating in research. In addition, work is progressing on identifying a site for a potential repository in a clay formation in China. The first project was completed at the end of 2018 and accepted by the China Atomic Energy Authority in October 2019. Two potential sites for further investigation have been identified26”.
Phasing out nuclear
- Germany decided in the early 2000s to phase out nuclear power (Green-SPD coalition headed by Chancellor Schroeder since 1998), and then opted in 2005 to stop reprocessing spent fuel. It is thus left managing both the waste vitrified before 2005 and the spent fuel produced after that date. The Gorleben salt dome in Lower Saxony was selected to dispose of this waste at a depth of 800m, in a former salt mine. This project was scrapped due to strong opposition from some local residents (initially opposed to surface or subsurface storage of that waste) as well as Germany’s Green Party, which was pushing for a quick phase-out of nuclear power. Now that the nuclear phase-out has been agreed upon (decision made by Angela Merkel following the tsunami and nuclear accident in Fukushima, Japan in 2011), Germany must properly manage its legacy waste and resume its search for a site, but it has lost 30 years. The Bundesgesellschaft für Endlagerung (BGE), Germany’s federal waste management organisation, recently drafted an initial analysis of potentially suitable geologies – granite, clay and salt – and concluded that “240,000 square kilometres, or more than 54% of the country’s surface area, could be suitable for deep disposal27”. The next steps will be to organise a consultation with elected officials and residents and carefully analyse the specific confinement properties of the geological sites. However, as one journalist pointed out: “Paradoxically, the strongest opposition has not come from the Greens. The Green Party Minister-President of Baden-Württemberg, Winfried Kretschmann, has admitted that waste must go to the safest possible place. The northern state of Schleswig-Holstein has also agreed to accept the waste if need be, with support from the Greens, following a debate full of ‘anger and tears’ within the party while he was still energy and environment minister, said party copresident Robert Habeck. After the ‘Nuclear power? No thanks’ movement of the 1970s, the Green Party entered the era of responsibility”, he said”28.
The geological formations explored in different countries are the same and significant potential exists like in France (due to the very composition of the Earth’s crust):
- Salt in Germany and the United States;
- Granite in Scandinavia, Switzerland, Russia and China;
- Clay in Switzerland, Belgium, France and China.
Countries like France, Germany, China and the United States have abundant clay, granite and salt resources. In other words, as of today, there are no technical constraints regarding the geological disposal of nuclear waste29, even if the technology was to be massively deployed for climate reasons over the next two to three centuries. In that case, the main challenge to tackle would relate more to fuel resources, a concern that is driving accelerated research into fast neutron reactors in Russia, China and the United States. In all countries, the issue of political acceptability at the local and national levels plays an important role and it too must be considered.
The technological advances that could pave the way for sustainable use of uranium resources and the partitioning and transmutation of waste
See IAEA-NEA, Uranium 2020. Resources, Production and Demand, 2020, as well as previous editions (available at www.oecd-nea.org).
See CEA, “Advances in research on partitioning-transmutation and plutonium multi-recycling in fast neutron reactors”, June 2015. Also see CEA, “The CEA at the Heart of Great New Challenges. Annual Report 2015”, 2015, and Thomas Gassilloud, member of the National Assembly, and Stéphane Piednoir, member of the Senate, “Nuclear energy of the future and consequences of the abandoning of the ‘Astrid’ Generation IV project”, report prepared on behalf of OPECST, 8 July 2021, National Assembly, Report No. 4331.
See CEA, “Partitioning-transmutation of long-lived radioactive elements”, December 2012, and “Advances in research on partitioning-transmutation and plutonium multi-recycling in fast neutron reactors”, June 2015.
If the goal is to have nuclear contribute to the climate change fight in a significant and lasting way, then it will be important to free up uranium 235 resources over the long term: it is currently estimated that proved reserves and resources that could possibly be accessed at reasonable cost would suffice to keep the global nuclear fleet in operation for 250 years based on existing installed capacity using slow neutron reactors (light water), covering 10% of global electricity demand (60,000t of natural uranium a year and about 15 million tonnes of global reserves)30. If all the uranium 238 was used thanks to FNR technology, these resources would suffice for a few millennia (multiplying the potential of the 15 million tonnes by a factor of around 50 to 100), not counting thorium, which would add an additional factor of 3.
Among the different fast neutron technologies, the most advanced is the sodium-cooled reactor; like other fast neutron systems, it allows plutonium to be recycled, eliminating the dominant actinide in terms of potential radiotoxicity of long-lived waste, and also dominant in terms of thermal load and thus the surface area required underground for disposal. One can hope that this technology will be fully mature between the middle and end of the century.
After presenting an overview of the outlook for sodium-cooled fast reactor technology, we will look briefly at the research being conducted into partitioning and transmutation of the other long-lived radionuclides, fission products and minor actinides.
This review of the current state of research will conclude with a few comments about the other generation IV “fast reactor” technologies (lead-cooled, helium-cooled, molten salt) and the possible use (for transmutation) of proton accelerators, Accelerator-Driven Systems (ADS), and lasers coupled with subcritical research reactors.
a) Sodium-cooled fast reactors: transmutation of plutonium and reuse of uranium 23831
It is possible to use light water reactors and slow neutrons (“thermal”) to burn a small portion of the plutonium through mono-recycling, but these slow neutrons do not allow for easy fission of these heavy atoms which, therefore, capture the neutrons and produce the “poison” isotopes of the chain reaction (such as even-numbered plutonium isotopes). This difficulty results in “hardening” the light water spectrum (i.e. accepting faster neutrons) with greater plutonium enrichment in the core, which is quickly limited for safety reasons (fewer delayed neutrons and less water proportionately to reduce the slowing of the neutrons). In other words, fast neutrons are needed to fission the plutonium (including the even-numbered isotopes), and the minor actinides, as are reactors designed for that purpose, meaning ones that have a heat transfer fluid that does not slow the neutrons as is the case with water. Hence the investigation of sodium, lead and helium, which offer better performance from that standpoint.
When it comes to fast neutron technologies, sodium-cooled reactors are the most mature, including from an industrial standpoint, and with large or small unit sizes. France has robust industrial and scientific expertise (the best, perhaps, until the recent cancellation of the Astrid project): Rapsodie 40 MW 1967-1983, Phénix 250 MW 1973-2010, Superphénix, EDF-CEA in cooperation with ENEL and RWE, 1 200 MW, 1985-1997. Russia also has industrial skills. It currently leads the field in fast reactors with sodium-cooled (BN 800 and 600 MW, in planning BN 1 200 MW) and lead-cooled technology (lead-bismuth, BREST 300 MW lead project). Japan (Monju sodium-cooled 250 MW reactor which will be dismantled) has been investing in the field for many years via close cooperation with France in multiple related fields: sodium-cooled fast reactors, reprocessing and vitrification of HLW.
Japan was very involved in research and planning for industrial deployment, yet the process was put on hold in recent years as the country reassessed and scaled back its nuclear strategy in the wake of the Fukushima accident. China has a very ambitious research programme and is gradually making up for lost time with prototypes and industrial demonstrators for all fast reactor technologies slated for use over the next decade (including a 600 MW sodium-cooled FNR under construction). For India, sodium-cooled fast reactors are an important step in a long-term nuclear strategy that aims to make use of the country’s thorium resources.
The United States was a pioneer in the industry in the 1950s but never got to the industrial phase since it moved away from the closed fuel cycle in 1979 (decision made by Jimmy Carter). Nonetheless, the country remains at the top of the field scientifically with large government-run labs like Argonne, Idaho, Oak Ridge, Los Alamos managed by the United States Department of Energy (DOE) and the significant needs for small modular reactors (SMRs) to power military submarines and aircraft carriers as well as military bases. The country is relaunching a major research programme that includes encouraging initiatives in the private sector with innovative projects such as Natrium, a 350 MW sodium-cooled fast reactor developed with TerraPower (Bill Gates) and GE Hitachi –, and the Versatile Test Reactor (VTR) project overseen by the DOE that aims to have a research FNR built by 2026.
Sodium FNR technology will need to overcome two key obstacles to move into industrial development: first, achieve the same level of safety as the generation III reactors when it comes to preventing and mitigating serious accidents (core meltdown and risk of criticality-negative void coefficient); second, simplify the overall design to make operations more robust and bring costs down to a level closer to those of light water reactors (with Astrid, the goal was to reduce the additional investment costs to below 30%, which was still too high, and, more importantly, has still not been achieved). Paths exist to meet the safety objectives: develop small modular reactors (fast SMRs) or work on large core design (some avenues were explored as part of the Astrid project with the “low void effect core” design: insertion of fertile layers, “sodium plenum”). It seems possible that solutions will be found to these problems within the next few decades (by 2050) with robust international cooperation and specifications that clearly focus on three key objectives: safety (fusion-criticality), simplification-robustness and cost control, and adapted cycle equipment (fabrication, reprocessing and transport of fuel). France could play a central role in this effort.
b) Overview of research into partitioning and transmutation of minor actinides and long-lived fission products32
The transmutation of long-lived fission products (iodine 129, technetium 99, caesium 135) can only be done via the capture of neutrons (non-fissile radionuclides). This is practically impossible today (except for only a part of the technetium 99 and with recurring cycles continued over several generations of reactors): we do not know how to introduce targets with iodine in the reactor, and caesium 135 is always accompanied by its isotope caesium 133, which also absorbs neutrons and produces caesium 135 again. It would nonetheless be possible to complete the first partitioning step to place certain products like iodine 129 into specific matrices-packages (though their resistance would need to be guaranteed over the very long term).
The partitioning of minor actinides is also feasible and the transmutation of americium is accessible with sodium-cooled FNRs. It is effective only as long as multi-recycling continues over time (secular process) and does little to reduce the potential radiotoxicity of the glasses over the long term (due to the production of curium), though it does significantly limit their thermal output and would thus, over time, reduce the surface area required underground for storage of HLW by a factor of 2. This approach can only be applied once FNR technology has been mastered and deployed industrially, and it would pose additional radiation protection and thermic problems during the handling of fuels containing americium in different phases of the fuel cycle (fabrication, reprocessing, transport), problems that would need to be resolved. It is necessary to assess what could be lost in the near term in terms of radiation protection for workers relative to the long-term gains in terms of storage space. In addition to americium, the transmutation of curium would technically be possible and would help reduce toxicity over the long term, though this appears to be a particularly sensitive subject today due to issues around radiation protection, as gains would be small in terms of thermal load and thus the footprint of the repository.
As mentioned above, the transmutation of plutonium in fast neutron reactors is of interest for managing waste since it does diminish both the radiotoxicity and thermal load of the waste (and thus the repository footprint), but first and foremost, it accomplishes the more important goal of using the energy potential of plutonium and the full, considerable potential of natural uranium, including its isotope 238.
Transmutation, beyond that of plutonium, can be a way to:
- Reduce the dose at the potential outlets of a geological repository;
- Reduce the long-term potential radiotoxicity of waste;
- Reduce the thermal load of the waste disposed of, and thus the underground footprint of the cells required.
For the Cigeo project, doses at the potential outlets are inexistent up to a million years, and are at that point negligible compared to natural radioactivity (1,000 times below natural radioactivity for HLW, 100 times lower if all spent fuel was to be disposed of), and are linked to a few traces of fission and activation products (iodine 129, selenium 79, chlorine 36). Transmuting the minor actinides is thus of no interest (they remain captured by the clay) and transmuting the long-lived fission products would hold almost no benefits in health terms. Moreover, the technique of transmutation has not been mastered regarding fission products as of today.
In sum, there are no health benefits to reducing potential radiotoxicity if Cigeo is built. It may be of interest if long-term storage is adopted, to reduce health risks if surveillance-maintenance of the facility is abandoned at some point in future. The greatest gains in terms of reduced radiotoxicity are obtained with the transmutation of plutonium, and only if it is done quickly, i.e. if spent fuel (UOX and MOX) is reprocessed and multi-recycled in fast reactors without delay (otherwise, the radioactive decay of plutonium and its descendants produces long-lived and radiotoxic radionuclides like curium). Going further would require also transmuting the curium, with great precautions taken to protect workers (verifying that benefits outweigh risks).
If the goal is to reduce the thermal load of waste so it does not have to be spaced out in order to preserve the qualities of the rock in terms of confinement, then beyond transmuting plutonium, it is the transmutation of americium that could reduce the footprint of the repository by a factor of 2 over the long term. This will be the most accessible step once fast reactor technology has been mastered along with the transmutation of plutonium. Based on the geological potentials available, this does not appear to be an urgent priority for the moment.
c) The outlook for innovation with transmutation over the longer term: other generation IV fast reactor technologies and accelerators/lasers combined with fast subcritical reactors (ADS)
The generation IV fast neutron technologies susceptible of contributing to plutonium recycling and, over the longer term, the transmutation of some long-lived waste
- Fast reactors cooled with lead and lead-bismuth: this is the most advanced technology after sodium. The Russians are successfully using it in their submarines, and it will be interesting to track progress with their 300 MW lead-cooled Brest reactor prototype. We have an opportunity to expand our own expertise in Europe with the prototype of a subcritical reactor (lead/bismuth-cooled) planned in Belgium with the ADS Myrrha project (supported by the European Union). Some technological barriers remain, particularly regarding potential corrosion related to the lead-bismuth.
- Gas-cooled fast reactors (helium): very significant technological barriers must still be overcome (safety, fuel-core materials that can withstand very high temperatures). The CEA moved into this field of research in the early 2000s. The United States currently has an innovative GFR project funded by the DOE (Fast Modular Reactor 50 MW, General Atomics with industrial cooperation partners).
- Molten salt fast reactors (MSFR): this is a very novel technology in its principle. The fuel is “dissolved” in the coolant in the primary reactor loop, allowing for reprocessing in line with the reactor. This technology offers promising possibilities (for “burning” all actinides, including the thorium), but significant technological barriers remain both in terms of the safety concept to be invented for this new type of reactor, and the need for primary loop and fuel materials that can resist corrosion from the molten salt under irradiation. The CNRS has developed useful scientific expertise in this promising field, which remains in the conceptual stage for now.
Looking further into the future:
proton accelerators and lasers with fast subcritical reactors Spectacular progress has been made in recent decades in terms of tools for researching power density in the field of particle accelerators and lasers. These new techniques have encountered roadblocks on the way to industrial development, notably due to the need to go from transmuting one atom to transmuting a very large number of them. This is why the research projects in planning today combine proton accelerators or lasers with fast subcritical reactors via spallation targets that the protons/lasers strike to generate the necessary neutrons to sustain the chain reaction in the subcritical reactors. These systems are conceptually interesting as they would allow the transmutation of numerous radionuclides, which is what they are intended to do (they are not designed for producing electricity): indeed, these are “machines” dedicated to transmutation, as they could receive high concentrations of minor actinides in their core, which is difficult to do with “conventional” FNRs for safety reasons. The downside is that the systems are extremely complex, due to the fact that they require combining an accelerator/laser-spallation target-subcritical fast reactor, and very significant technological barriers must still be overcome (including with the subcritical fast reactor and its safety).
Through a European cooperation project, Belgium is leading the Accelerator-Driven System (ADS) Myrrha project, in which France is participating, coupling a proton accelerator with a lead-bismuth fast reactor. French laser specialist Gérard Mourou, who was awarded a Nobel Prize in Physics in 2018, has proposed some interesting research ideas involving lasers. These systems have little chance of becoming mature in the very near future, but this is a fertile field of research that deserves to be closely followed.
In sum, much is at stake in the quest to bring these new technologies to market (from fast reactors to ADS) and make them competitive: they would allow the nuclear power industry to massively produce dispatchable energy with no CO2 emissions for many centuries, without any fuel sourcing issues, guaranteeing security of supply for France and better management of long-lived waste. This is undoubtedly why Russia and China are moving into high gear, and the United States, after a long hiatus, is promoting public-private research projects in nearly all the promising fields listed above. This will also be an important undertaking for France and Europe in terms of scientific expertise and their sustained contribution to carbon neutrality and industrial and energy independence. If these objectives are to be met, then the first step that must be taken, and probably the most useful one, is to complete FNR development (sodium being the top candidate) so that plutonium and depleted uranium can be burnt. It should also be noted that starting fast neutron reactors depends on being able to mobilise the plutonium from spent fuel from PWRs, which may help explain why some countries that have opted for an open fuel cycle are hesitant to place it with spent fuel in geological repositories that are designed to be closed or to use it in PWRs through multi-recycling.
Looking more specifically at long-lived waste management, it is clear that, no matter how much (desirable) progress is made, there will still be long-lived waste to manage (though quantities may decrease going forward), starting with existing ILW-LL and vitrified waste that it is almost impossible to transmute. In other words, the prospect of progress with transmutation does not eliminate the need to make a choice between geological disposal and long-term storage.
No comments.